Demand-Pull Tools for Innovation in the Cement and Iron and Steel Sectors
In this report, RFF researchers explore the opportunities for applying relatively underused demand-pull policies to decarbonize the iron and steel sector and the cement sector.
Executive Summary
Demand-pull measures are a set of relatively underused policies for driving innovation by increasing the demand for innovative technologies. These measures contrast with supply-push policies, such as research and development funding, that subsidize the supply of innovative technologies. Demand-pull policies include:
- Prizes for innovation
- Advance market commitments (AMCs), which commit to purchase innovative products when developed
- Government procurement, where the government provides additional demand for innovative technologies
- Milestone payments, where funding is released in stages at the completion of various development stages
- Standards, which can stimulate demand by providing information about technology performance
- Contracts for differences, which can reduce price volatility-related risk related that can hamper innovation
Literature Review
We performed an extensive literature review for each of these policies, particularly looking at their applicability to various stages of innovation. The results of this review are summarized in Table 1.
Here, technology readiness level (TRL) gauges the maturity of a particular technology, with lower numbers (1–3) being early-stage research and development, middle numbers (4–6) being pilot stage, and the largest numbers (7–9) being demonstration and commercial deployment. Another important aspect not reflected in the table is that the size of the monetary reward must be comparable to the scale of the goal. This is particularly important for demonstration projects in the energy sector, which can cost over a billion dollars.
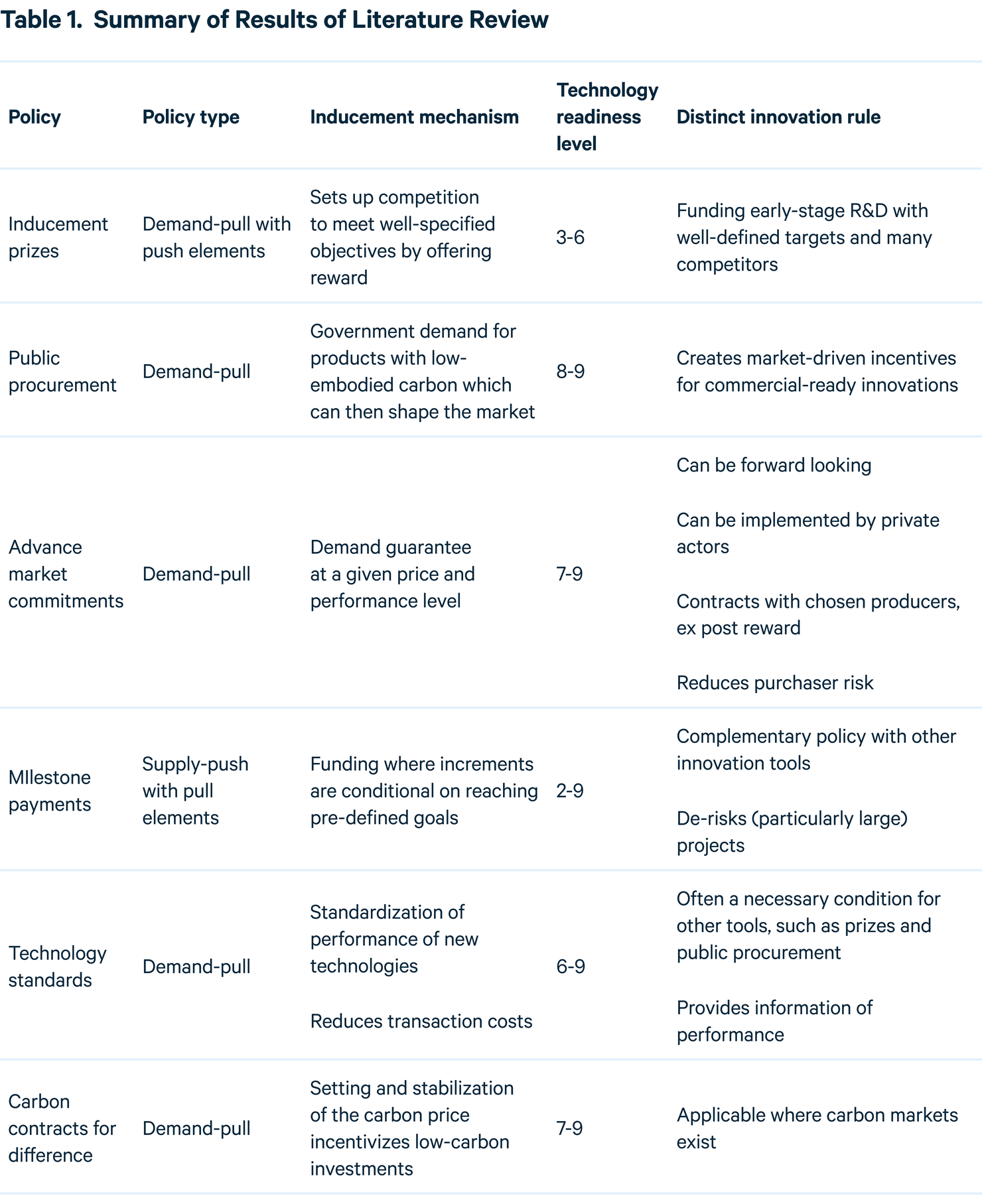
Recent Legislation
While there has been a welcome increase in the use of these policies in recent years, there is still room to increase their use, particularly in the context of low-carbon technologies. The passage of the Infrastructure, Investment, and Jobs Act (IIJA) and the Inflation Reduction Act (IRA) has led to the creation of the Office of Clean Energy Demonstrations at the Department of Energy (DOE), which released a request for information on the use of demand-pull policies in February 2023.
These bills appropriated billions of dollars for demonstration funding by DOE. However, they also included a number of demand-pull policies. These include:
- Prizes for direct air capture
- Government procurement of clean building materials as part of the Buy Clean Initiative
- Carbon intensity standards for concrete
- Procurement of carbon from direct air capture (in the Omnibus Appropriations Act)
In addition, milestone payments are incorporated in many of the demonstration funding programs by DOE under the IIJA. For example, the hydrogen hubs program releases the funding in four stages, with goals to be met at each stage of the project.
Application to Steel and Cement
We next explore the opportunities for applying demand-pull policies to the iron and steel sector and the cement sector. In each sector, we consider the technologies needed for its decarbonization, the current policy roadmap, and the applicability of demand-pull policies as complements (or substitutes) for current policies. We also separately look at crosscutting technologies such as carbon capture and low-carbon process heat that can help decarbonize these sectors.
Steel Production
The main technologies to decarbonize steel production use hydrogen and electrolysis. Hydrogen steelmaking is already being demonstrated in the HYBRIT project, a Swedish joint venture producing fossil fuel-free steel with hydrogen direct reduction of iron to replace the traditional coal-based technology, and the electrolysis technologies are in the pilot stage. Given that these technologies with high TRL exist and have the potential to almost completely decarbonize the sector, funding for demonstration projects is likely the most important funding mechanism. There may be opportunities to enable use of lower-quality steel in electric arc furnaces. AMCs and government procurement are demand-pull tools that could also help deployment, but given the multi-billion dollar cost of a single steel plant, large sums of money would have to be devoted to such mechanisms. With respect to prizes, they work best with multiple competitors, and the steel industry is highly concentrated in the United States.
There may be opportunities for AMCs along the value chain, such as in intermediate, fabricated steel products (such as sheets, plates, bars, beams, pipes, and tubes) due to the large number of promising technologies and a considerably higher degree of diversity in final products that use steel. Government procurement, while likely not sufficient to drive deployment of zero-carbon steelmaking by itself, can still provide a valuable source of revenue for producers. Carbon intensity standards are being developed to enable procurement under the Buy Clean Initiative.
Cement
Unlike iron and steel, where the technologies are at higher TRLs, low- to zero-carbon cement is still in the research and development stage. We find that the development of a low- to zero-carbon cement has many of the characteristics that make the use of prizes beneficial, such as low TRLs, product uniformity, and existing emissions reporting systems.
Specifying a prize for cement will require a detailed set of material standards to ensure the material can be widely used as a substitute for current cement uses. There would also need to be a specification for the amount of carbon involved in the creation of this cement. Given the need to achieve net-zero carbon emissions by the second half of the century, we suggest that the standard for carbon emissions be a very high percentage reduction from current carbon intensities. Another choice is whether to focus on all embodied emissions in the concrete sector or to focus on the process emissions. We suggest that the standard focus on the process emissions, since those are the emissions fundamental to cement creation and that cannot be abated in any other manner. As there are proposed alternate cements that absorb carbon dioxide as they cure, removing carbon from the atmosphere, these negative emissions should be included in any carbon accounting.
Finally, any such cement substitute must be deployable at scale. In particular, this means that any feedstocks into the production process must be relatively inexpensive and available in large quantities. Similarly, the price differential as compared to traditional cement, perhaps expressed in terms of an implicit carbon price, must be reasonable.
Because AMCs can be made for future products, they may be more applicable to demonstrate low-carbon cement technologies as they progress beyond pilot scale. While individual cement plants can be quite expensive, the overall scale of cement use in the United States means that there is likely enough capital to enable sufficiently large AMCs to be effective.
The development of requirements for such a prize or AMC is a substantial endeavor in itself. For instance, the value of the prize or the price of the AMC must be determined, balancing the cost to the government with the need to incentivize the research and benefits of success. We believe that the benefits of a low-carbon, scalable cement are substantial, which would justify a relatively large prize or market commitment.
Carbon Capture and Process Heat
Carbon capture, utilization, and storage (CCUS) and zero-carbon process heat are technologies that can be deployed across a wide array of sectors and are also applicable to steelmaking and cement manufacture. However, because neither of these technologies are products that anyone purchases, it is not immediately clear whether a demand-pull policy is desirable. One could use prizes or AMCs for products produced using CCUS or zero-carbon process heat, but it would likely be more efficient to instead set a standard for the carbon content of the final product and then be agnostic with respect to how the manufacturing process achieves that standard. One exception to this may be milestone payments, which, because they can apply at lower TRLs, could be used for funding more nascent carbon capture and process heat technologies.
Conclusions
DOE faces huge challenges to meet its new decarbonization goals. In a tight labor market, it needs to hire people knowledgeable about CO2 mitigation and efficient decisionmaking. Even with all the cash flowing and subsidies, its grantees may be reluctant to propose and promise success for massive projects with unproven (at scale) technologies and with private funding hard to come by. By focusing on boosting demand, demand-pull policies can reduce offtake risks, making private capital financing a better bet.
However, these tools will not implement themselves. Green procurement, for instance, requires carbon intensity benchmarks, environmental product declarations, and decisionmaking tools that need knowledgeable staff and time and resources to learn how to design and use them. Early failures, in the face of all the pressure to succeed quickly, need to be expected and tolerated. Legal and administrative barriers may also limit the application of some tools we reviewed, although the relevant agencies have used some types of these tools before. Ultimately, greater reliance on these tools requires high-level administrative commitment and, perhaps, a willingness to be patient.
As with more traditional supply-push policies, demand-pull policies inherently involve picking winners and will be less efficient than policies that cut across sectors and provide medium- and long-term incentives to reduce greenhouse gas emissions. Such policies as carbon taxes, tradable performance standards and other regulatory approaches have recently been off the table legislatively. But they need their day in the sun, as well.
1. Introduction
Meeting the US net-zero greenhouse gas (GHG) emissions goal by 2050 will require massive reductions in emissions from all emitting sectors. While the power and transportation sectors are responsible for a large share of GHG emissions, the industrial sector comes in third, with annual emissions of 1,521 million metric tons (Mt) in 2019, one-quarter of US GHG emissions (EPA 2022). The industrial sector is expected to become the largest set of sources by the next decade, with the top emitters being steel, concrete, chemicals, and refineries. These industries are hard to decarbonize because their capital stock is expensive and turns over slowly, they often need very high heat sources, and many have process emissions, meaning that the emissions are an inherent part of the chemistry and cannot be abated by fuel substitution.
To bring about carbon reductions in this sector, government policies will need to require or otherwise incentivize making such reductions. These policies (defined loosely) include planning documents, such as the US Department of Energy’s Industrial Decarbonization Roadmap (DOE 2022d), as well as state and private decarbonization roadmaps; regulatory and tax subsidy policies (the sticks and carrots); and a variety of policies that directly stimulate innovation as either supply push —offering money to innovators in the form of grants or low-interest and guaranteed loans—or what we refer to as demand pull policies, a relatively underutilized class of innovation policies such as prizes, advance [JB7] [LB8] market commitments, green procurement, and contracts for differences. Some policies may not directly be innovation policies but may work through building demand to obtain innovation. We classify those by their main characteristic.
This paper is about whether such demand-pull policies offer advantages over (or can complement) supply-push policies for stimulating innovation in the cement and steel industries in the context of DOE innovation policies, as well as those of the US Environmental Protection Agency (EPA) and US General Services Administration (GSA) for green procurement. We focus primarily on DOE, however, because of its long history in supporting research, development, and demonstration (RD&D) and because it is the focus of almost all new government spending for industrial decarbonization.
We start in Section 2 by discussing the innovation process and the technology readiness scale that figures heavily in DOE’s work and in this paper. In Section 3, we describe the full set of tools affecting innovation, including supply-push and demand-pull innovation policies, as well as regulation, tax subsidies, private R&D programs, and several other categories, pointing out the pros and cons of each. This section is partly based on a detailed review of the academic literature and includes comments on practical experience with these policies. In Section 4, we examine recent trends in government RD&D, including in demand-pull policies. In Section 5, we explore the opportunities for applying demand-pull policies to the iron and steel sector and the cement sector. For each sector, we consider the technologies needed for decarbonization, the current policy roadmap, and the applicability of demand-pull policies as complements (or substitutes) for current policies. We also separately look at crosscutting technologies, such as carbon capture and low-carbon process heat, that can help decarbonize these sectors. Section 6 concludes with suggestions for the role of demand-pull policies going forward.
2. Stage Setting
2.1. Different Stages of Innovation
There are many ways to classify the innovation process, but for the purposes of this paper, we use DOE’s Technology Readiness Level (TRL) scale (DOE 2011). This scale, shown in Table 2, was originally developed for use by NASA and adopted by DOE for use in Technology Readiness Assessments. The TRL scale indicates the maturity level of a given technology and ranges from 1 (basic principles) to 9 (total system used successfully in project operations). Other TRL scales exist, of which the most prominent was developed by the International Energy Agency, whose scale has 11 points and refers to both technological development and market adoption (IEA 2020b).
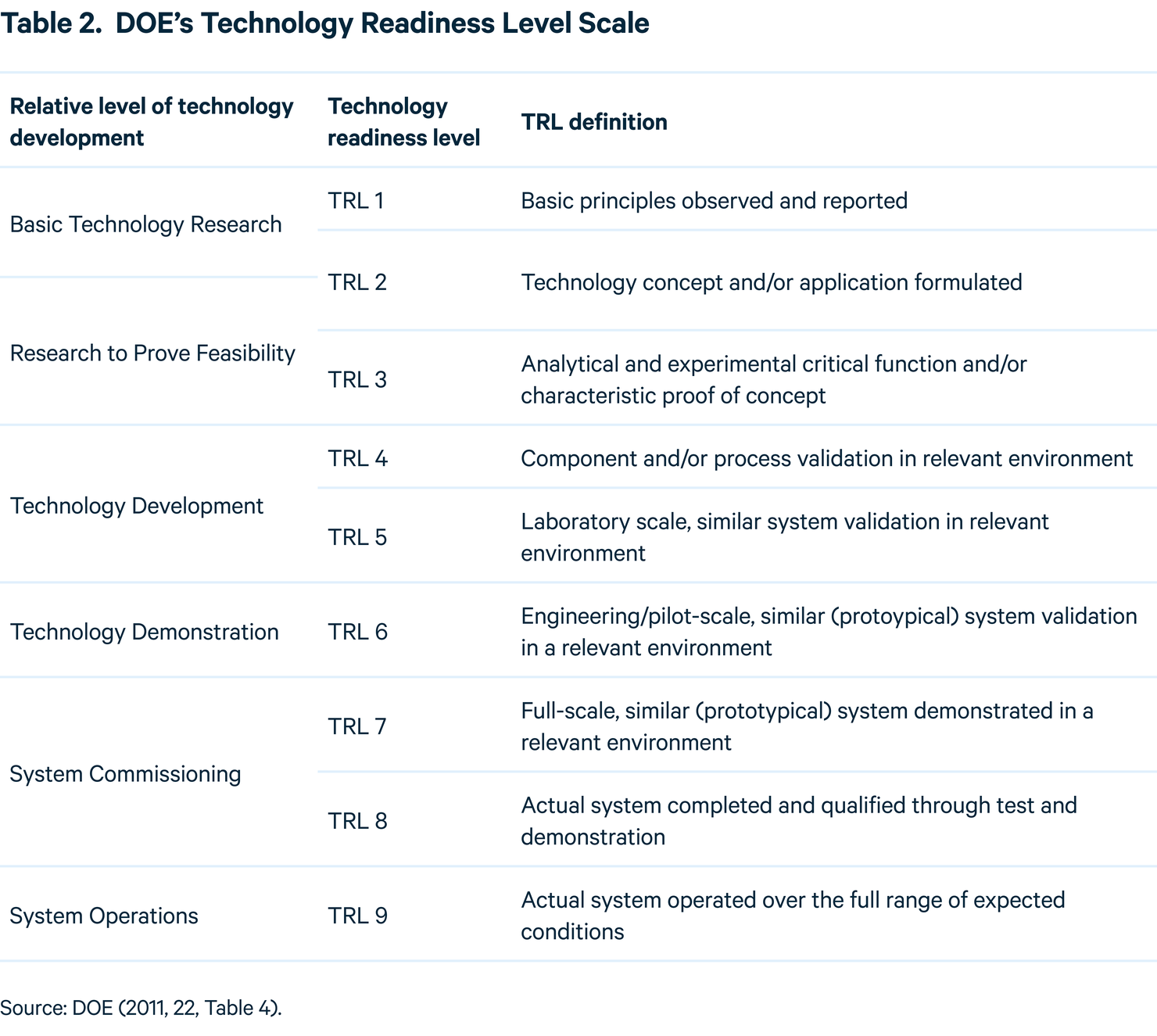
At the lowest levels of the DOE scale is the Basic Technology Research (TRLs 1–2) phase. In this phase, scientific research is translated into a technology’s basic principles and speculative practical applications, and the goal is to begin determining potential use cases through analytical studies. Next is the Research to Prove Feasibility (TRLs 2–3) phase, which includes additional applied research along with laboratory-scale studies. This phase is composed of analytical studies along with laboratory-scale studies that can help validate hypothesized use cases. The basic components are then integrated into a laboratory-scale system to establish that they work together in the Technology Development (TRLs 4–5) phase. This system configuration is likely to be similar to the eventual application of the technology.
In the Technology Demonstration (TRL 6) phase, the technology moves beyond the lab, and engineering-scale prototypes are tested in a relevant environment. This is akin to a pilot phase and is a significant step up from the laboratory work in previous phases. From this phase onward, projects likely require partnerships with the private sector to further validate the technology. This is followed by full-scale testing in the System Commissioning (TRLs 7–8) phase, which marks the end of system development for the technology. In this phase, the technology undergoes rigorous testing as a full-scale prototype. The goal is to prove that the technology can function under expected conditions (e.g., with real feedstock under certain weather conditions). In its final form, the technology reaches the System Operations (TRL 9) phase and can operate under a full range of conditions. Technologies that reach this phase are expected to be fully commercialized and capable of operating at scale.
Overlaid on this scale is a concept called the “innovation valley of death” (Figure 1). This term describes the point in the technology development process at which basic research has proven out and the projects are ready to be developed into pilots and prototypes, but university and government funding typically peters out, while venture capital and other private capital assess the investment risk as too high. This issue is especially relevant in the energy technology area, where capital requirements for demonstrations are high and development timelines are usually longer than traditional venture capital’s timelines. Thus good ideas may not make it out of this valley without purposeful programs to derisk projects, such as through targeted government innovation programs (Nemet et al. 2018).
Figure 1. The Innovation Valley of Death
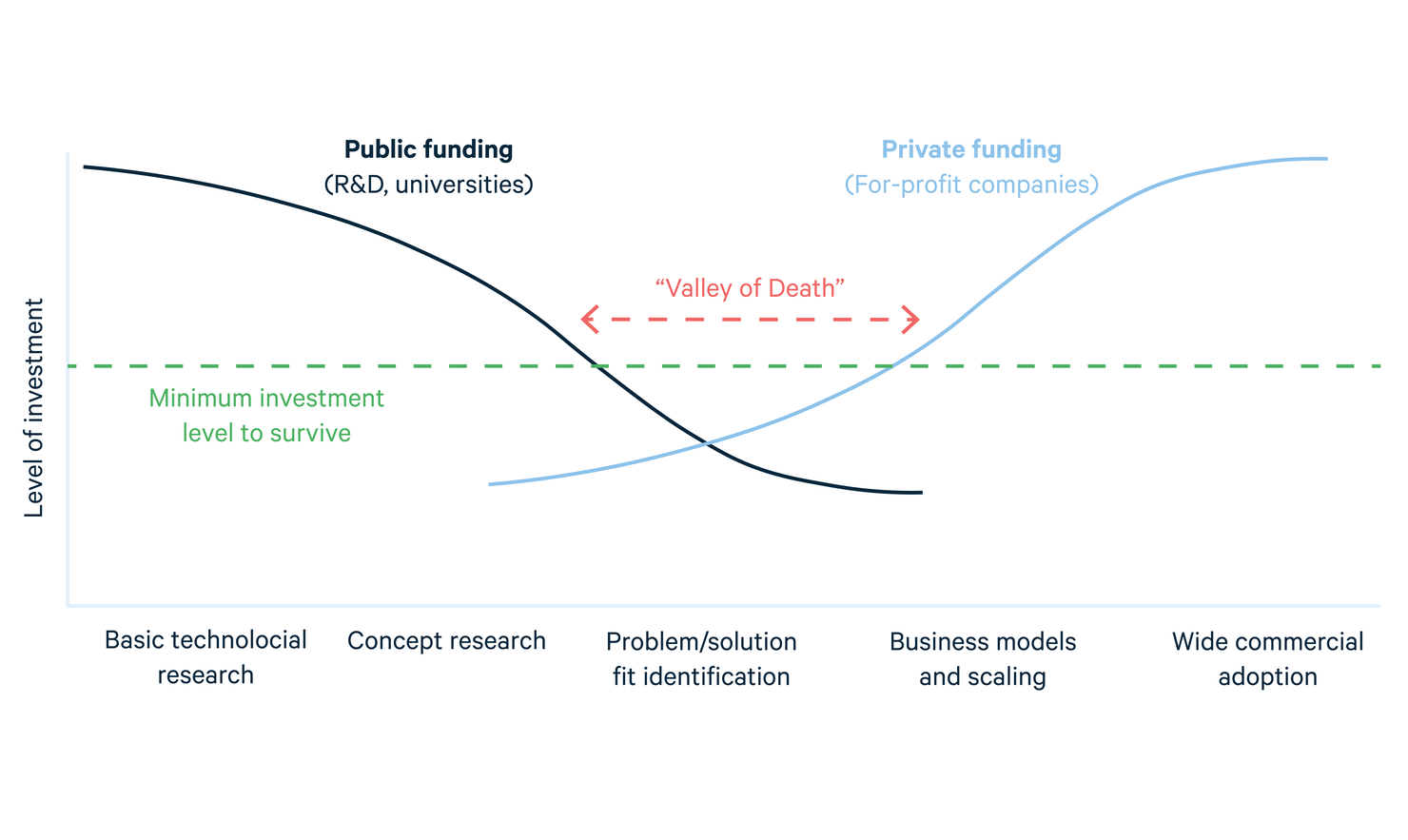
Source: Skillicorn (2021)
Certain offices at DOE focus efforts on specific categories of the TRL scale (Figure 2). For example, basic science research (TRLs 1–3) is explicitly supported by the Office of Science, while the Advanced Research Projects Agency–Energy (ARPA-E) and the Small Business Innovation Research and Small Business Technology Transfer (SBIR/STTR) programs also fund lab-scale prototyping (TRLs 2–6 and 1–5, respectively). On the other end of the scale, the Loan Programs Office (LPO) supports commercial-scale deployment projects that have advanced beyond the development phase (TRLs 8–9). The Office of Clean Energy Demonstrations (OCED), created in 2021, bridges the gap in funding between programs focusing on technology developments and LPO funding technology deployments. It helps innovations escape the valley of death by supporting large-scale demonstration projects. Both OCED and LPO are multitechnology offices that have authorization to fund projects that span the decarbonization landscape. LPO, through loan guarantees and financing in support of or in lieu of private sector financing, focuses primarily on initial commercial deployments, whereas OCED provides support for projects that are beyond the early-stage demonstration phase but are still too risky for full-scale commercial deployment. Each can fulfill a vital role in bridging the valley of death.
Figure 2. Technology Readiness Level Focus of DOE Office
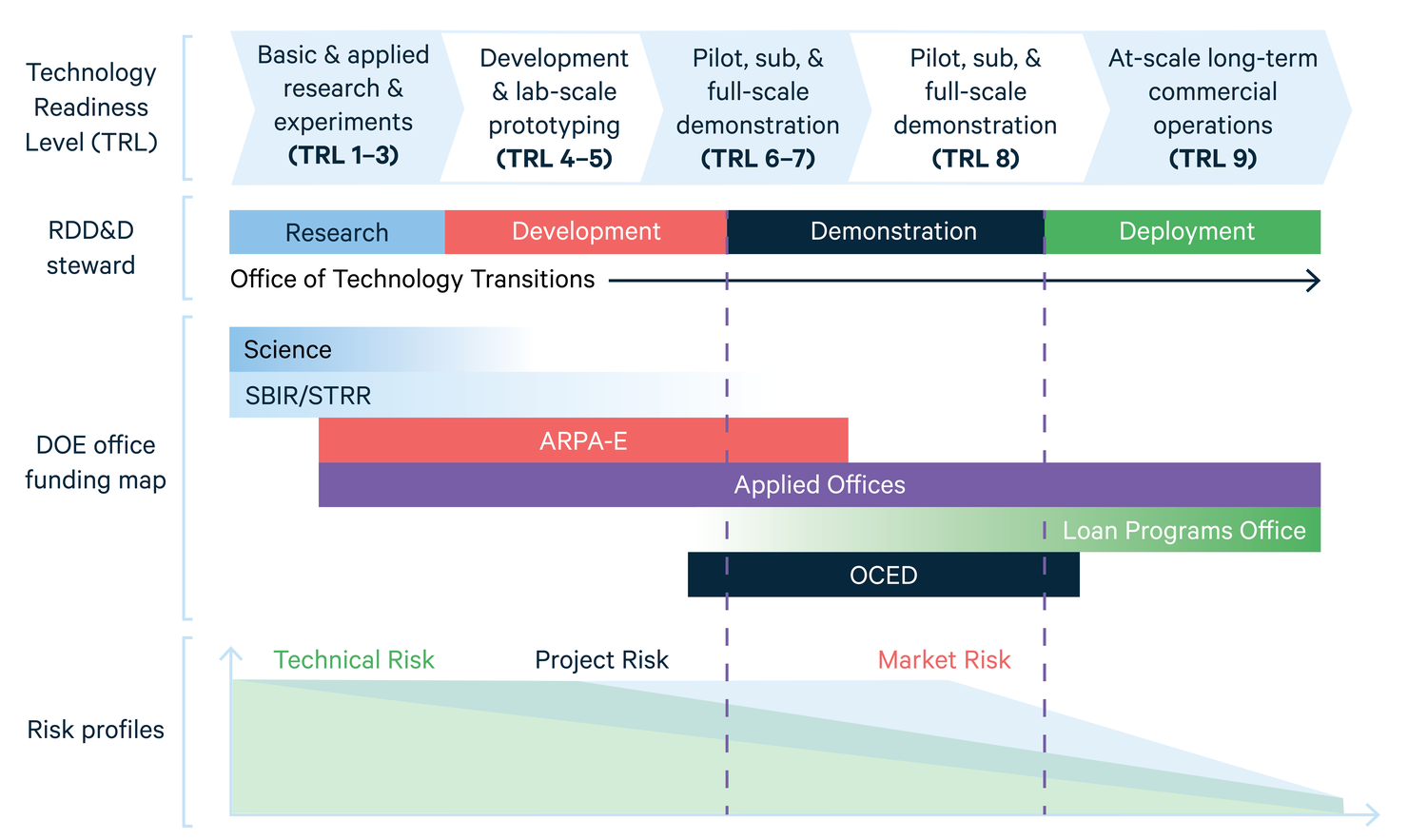
Source: Walrond (2022).
Most of DOE’s technology offices involved in advancing industrial decarbonization efforts have activities covering the entire TRL scale (Figure 3). Historically, industrial decarbonization activity has mainly taken place in the Advanced Manufacturing Office (AMO) in the office of Energy Efficiency and Renewable Energy (EERE), but other offices have also played important roles, such as the Office of Fossil Energy and Carbon Management (FECM) for carbon capture technologies. In 2022, AMO reorganized and split its RD&D funding between the newly created Industrial Efficiency and Decarbonization Office (IEDO) and Advanced Materials and Manufacturing Technologies Office (AMMTO). Priorities within these subprograms can range from earlier-stage R&D in partnership with national labs and universities to later-stage pilot and demonstration projects. Similarly, FECM has an array of programs that fund both new technologies and demonstration of more proven approaches. For example, FECM’s Point Source Carbon Capture Program, which prioritizes the cement, steel, and hydrogen production sectors, is focusing on new capture technologies in addition to technologies in its portfolio that are in the demonstration phase. While these offices have primarily relied on traditional RD&D funding through funding opportunity announcements (FOAs) and grants, they also have a limited number of creative funding mechanisms, such as prizes, other forms of competitions, and technical assistance. We use the term grants to include other forms of contracting, such as cooperative agreements.
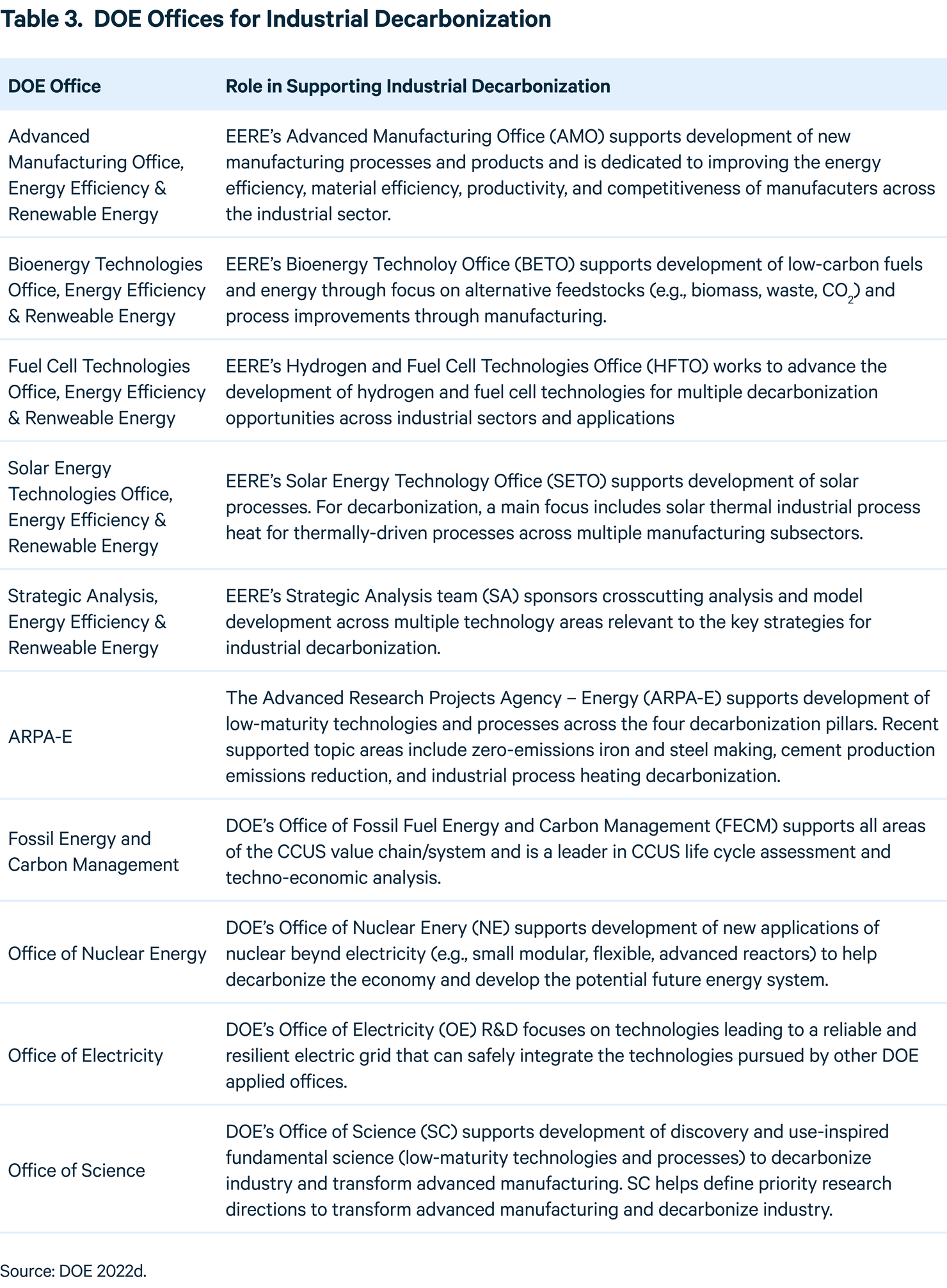
Source: DOE 2022d.
Other offices, such as the Bioenergy Technologies (BETO), Hydrogen and Fuel Cell Technologies (HFTO), and Solar Energy Technologies (SETO) Offices, all within EERE, as well as DOE’s Office of Nuclear Energy (NE), allocate some funding specifically to scale, pilot, and demonstrate technologies that are farther along the TRL scale. This is typically in addition to several other RD&D initiatives within these offices that are TRL-agnostic. BETO and HFTO both have System Development and Integration subprograms, which work on the development, testing, and verification of engineering-scale R&D projects. HFTO supports the Hydrogen Shot initiative and seeks to demonstrate hydrogen’s role in end uses such as energy storage, industrial and chemical manufacturing, and transportation. SETO focuses on strengthening manufacturing and value chain capacity, and its American-Made Solar Prize supports the launch of new products with a focus on domestic manufacturing. The Advanced Reactor Demonstration Program , run jointly by NE and OCED, does not directly fund demonstration projects, but rather serves as a complement to OCED’s multiyear funding for nuclear reactor demonstration and commercial deployments. This program funds later-stage R&D and complementary elements necessary to further derisk these deployments, with four pillars focused on innovation, risk reduction, regulatory development, and safeguards.
In general, DOE’s technology offices have flexibility as to which stage of the TRL scale their subprograms and initiatives target. Over time, funding requests for some subprograms may increase once new technologies become more proven and a shift toward higher-TRL activities is necessary.
LPO focuses on high-TRL technology ready for deployment and diffusion. It provides funds through five programs: the Title 17 Innovative Clean Energy Loan Guarantee Program, Advanced Technology Vehicles Manufacturing Loan Program, Tribal Energy Loan Guarantee Program, Carbon Dioxide Transportation Infrastructure Finance and Innovation Program (in partnership with FECM), and Energy Infrastructure Reinvestment Program.
OCED focuses on a slightly lower TRL than LPO, where there is still a high technological risk. Its portfolio includes the following programs: Advanced Reactor Demonstration Projects, Carbon Capture Large-Scale Pilot Projects, Carbon Capture Demonstration Projects, Clean Energy Demonstration on Current and Former Mine Land, Energy Improvements in Rural or Remote Areas, Industrial Demonstrations, Long-Duration Energy Storage Demonstrations, Regional Clean Hydrogen Hubs, and Regional Direct Air Capture Hubs .
Additionally, as discussed in Section 4, recent legislation includes major funding provisions for OCED and the technology offices that could address needs in higher-level TRL activities. Of the $62 billion appropriated to DOE from 2022 to 2026, $41.7 billion could be devoted to RD&D (DOE 2021; Chong and Hart 2022). As a result, changes may occur in the technology offices’ programs, with more RD&D funding allocated explicitly for pilot projects and demonstrations.
2.3. Decarbonization Innovation outside of Government
While governments make funds available for innovations, whether on the demand or supply side, the private sector usually spends sizable sums on innovation and then, to more fully realize the fruits of such innovations while operating in a competitive market, uses the patent system to protect its intellectual property. From a decarbonization perspective, however, unless an innovation adds to profitability (by adding to revenue or reducing costs), it is unlikely to be made or implemented by the private sector. So industry is unlikely to invest in decarbonization innovation without regulation, government funding, or some other inducement to do so.
Nevertheless, decarbonization is often a joint product with profitable technologies, such as those delivering energy efficiency, which both decreases energy bills and reduces CO2 as a result of burning less fossil energy. The huge reductions in CO2 emissions from substituting cheaper natural gas for coal in power generation are another example of how greater profitability can lead to major CO2 reductions in the absence of regulation. Firms are known to be strategic about their RD&D spending, realizing that future regulations may be preempted or weakened if emissions reductions can be delivered “early.” Moreover, as a fairly recent phenomenon, shareholders, boards of directors, rating agencies, and lenders all put pressure to varying degrees on industry to be a good corporate citizen—for instance, to set and implement goals for reducing greenhouse gas emissions within the firm. Thus some degree of emissions-reducing activities will occur in the absence of regulation or government-supported innovation funding. But this private action in the absence of government regulations or incentives is extremely unlikely to produce the level of emissions reductions needed to achieve national climate goals.
Besides the industrial and financial sectors, other elements of the broad private sector are active in seeking to decarbonize industry, including the tech and other relatively clean sectors and private foundation spinoffs. One major development in the tech sector has been led by Stripe, Meta, Alphabet, McKinsey Sustainability, and Shopify, which have entered into a partnership to offer advance market commitments (a form of demand-pull mechanism discussed in Section 3.2.2.3) with companies developing permanent carbon removal technologies and facilities. The Frontier Fund, formed to oversee this task, is capitalized at almost $1 billion to obtain CO2 reduction credits that the fund contributors will use to meet their own net-zero CO2 goals while making credits available to other businesses (Frontier 2022). While the targeted technologies, such as direct air capture, are not strictly industrial decarbonization technologies, their maturation could mean that industry might meet its goals in part through purchase of such credits. The green foundation community has also been seeking to decarbonize industry and created a number of venture capital spinoffs for funding the development of decarbonizing technologies. One of the most prominent is Breakthrough Energy Ventures fund.
3. Policy Options for Incentivizing Innovation
In this section, we consider why tools to stimulate innovation are needed and review the types of tools available. We describe both supply-push and demand-pull policies and provide a summary of the literature on demand-pull technologies. Finally, we briefly discuss regulatory measures (sticks) and tax credits (carrots), which can have an ancillary benefit of inducing innovation.
3.1. Motivation for Innovation Policy
Government intervention is justified in the presence of uninternalized externalities. Within the area of industrial decarbonization, the obvious uninternalized externality is greenhouse gas emissions, which lead to damaging climate change. Internalizing this externality would have two important effects. One is that it would incentivize polluters to reduce their carbon footprint to the point where their tax payment and marginal abatement costs are equal. In the short term, these costs may be large, given existing GHG-reducing options. The other, in the longer term, is that avoiding the tax would provide incentives to the market to develop and implement new technologies.
In addition, the market for innovations and the process of bringing them to commercialization have their own market failures. One is a lack of appropriability. The patent system exists to provide innovators the ability to profit from their innovations for an extended period of time without direct competition. But minor changes in technologies can skirt patent protection, and in the longer term, the patent ends, diminishing the ability of innovators to appropriate their ideas. Financial markets also have their own failures, such as the valley of death discussed in Section 2.1, a period in an innovation life cycle between small pilots and commercialization in which finding private finance is difficult. Thus both regulatory and innovation policies can be justified by market failures.
Recognizing these failures, the tax code permits R&D expenses to be deducted against revenues, after they are capitalized and amortized. This reflects a tightening of tax rules, which had permitted all R&D to be expensed. Further, an R&D tax credit can be taken for truly innovative projects (around 6 to 7 percent), and start-ups with little tax liability can take the credit against payroll taxes.
3.2. Types of Innovation Tools
This section describes a set of policy instruments that can be used to bring about innovation in the industrial sector. We classify each instrument as either supply push, meaning it targets increasing the supply of innovations, or demand pull, meaning it augments the demand for innovations. This definition differs slightly from that used by Nemet et al. (2018), who classify supply-push policies as those that reduce the cost of innovation and demand-pull policies as those that increase the returns to innovation. The two types of policies complement one another, although in practice, either one type or the other is often used. Moreover, some types of demand-pull policies are more appropriate for lower-TRL innovation and others for furthering penetration of high-TRL technologies.
For each instrument, we performed an in-depth literature review to find theoretical and empirical evidence regarding the conditions in which such policies could succeed. In Table 4, each policy instrument listed in the first column appears in a single row, with its main characteristics and some preliminary conclusions on applicability to the industrial sector. The second column indicates whether each instrument fits into a supply-push or demand-pull logic, and the third describes the mechanism that incentivizes innovation. The TRLs in the fourth column denote the stage of the innovation process in which the policy would be the most relevant. The fifth column identifies the policy’s distinct innovation role, or its particular niche. The evidence for the success of a given instrument from the theoretical and empirical point of view appears in the sixth column. The second column from the right describes the potential application of each innovation tool to the industrial sector. Finally, the last column presents potential challenges, barriers to the success of each instrument.
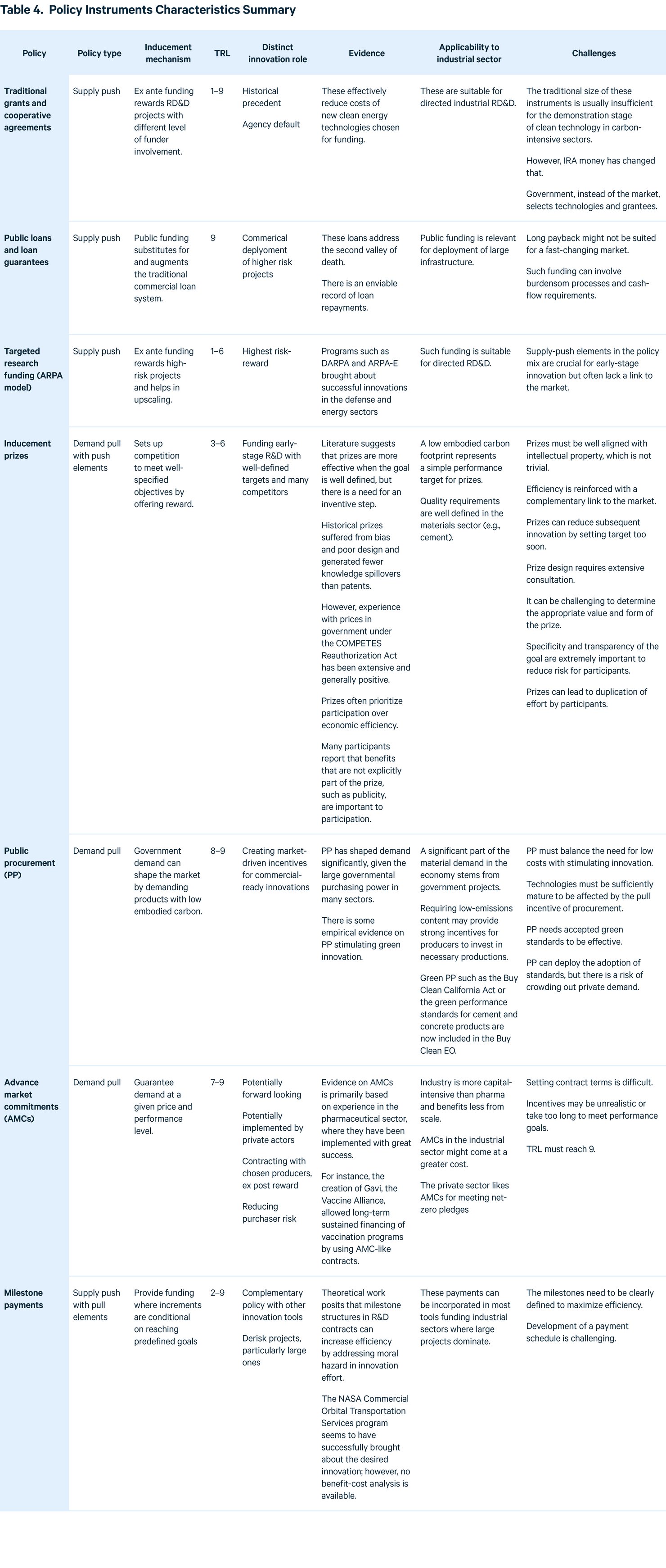
3.2.1. Supply-Push Policies
DOE’s primary tools for stimulating innovation are competitive grants, low-interest loans, and loan guarantees, which we classify as supply-push policies because they target innovating companies and other institutions, as opposed to creating demand for such technologies or the products they help produce.
3.2.1.1. Traditional grants and cooperative agreements
Most of DOE’s innovation spending is through traditional grants and cooperative agreements. Existing literature finds many positive benefits of these programs. For example, traditional RD&D programs can effectively reduce capital costs of new clean energy technologies. This is in part due to the increase in technological experience and knowledge stock in the form of technology diffusion (Samadi 2018). As experience with new technologies and processes increases, especially with smaller-scale modular technologies, costs may decline. Additional work has been done to quantify the value of this funding on the creation of new patents (Myers and Lanahan 2022).
However, these necessarily involve the government choosing which projects to fund and can come with strings attached, such as a requirement for environmental justice and community benefits plans to be submitted with the application. Many DOE funding opportunities target innovation at the lower end of the TRL scale (TRLs 1–5). At higher TRLs, energy technologies are often complex, integrated systems with numerous individual components, which require piloting, demonstrations, and integration with existing systems (Hart 2017). Thus traditional grants with smaller dollar values may have limited effectiveness for higher-cost technologies seeking to cross the valley of death.
3.2.1.2. Loan guarantees and low-interest loans
Loan guarantees are another tool that DOE has for supply-push innovation policy. DOE was first authorized to make loan guarantees in the Energy Policy Act of 2005, but the LPO’s first loan was not until 2009. Since then, the office has issued over $35 billion of low-interest loans (benchmarked at the relevant US Treasury rate plus a spread) and loan guarantees to more than 30 projects that either sought their first commercialization of decarbonization investment or were farther up the innovation process to full market acceptance. LPO also supplies expertise to its borrowers.
LPO’s programs address the part of the valley of death at which technologies move beyond the prototype phase and toward deployment; some call this the “second valley of death” (Hart 2017). In energy innovation, the costs and risks of demonstration are often higher than in other fields and have longer development times, discouraging private investors (Hart 2017). By funding the initial demonstration of these technologies, the LPO serves an important role in derisking these investments—giving private investors greater comfort with planned projects.
As with any of the supply-push approaches, a common criticism of the LPO is that winners are picked instead of being selected through a market-based mechanism (Bhandary et al. 2021). Specific to LPO, only certain types of innovations fit into its programs, and they must meet a threshold size. Interviews conducted by Bhandary et al. (2021) have noted that project developers were uncertain about their eligibility. Because of the lack of transparency, confusion arose around the selection of recipients that were large firms rather than smaller ones. This has raised the concern that this program seems to favor large corporations over smaller firms. Moreover, some potential applicants “were discouraged by the time-consuming process and high transaction costs involved” (Bhandary et al. 2021). In the past, project developers seeking loan guarantees paid a risk-based credit subsidy cost at closing. Authorizations in 2021 and 2022 from the Infrastructure Investment and Jobs Act and Inflation Reduction Act (discussed in Section 4.1) include provisions that should help streamline the application process, along with associated fees, and also set aside $3.6 billion to cover the credit subsidy cost of loan guarantees (Brasher et al. 2022). However, other requirements, such as the community benefits plan, have been added, which may increase the time and cost burdens on project developers.
Besides these obstacles during project selection, further challenges arise for selected projects. As in any program, the loan must be repaid on a defined schedule. Furthermore, loan agreements typically have covenants that may require project developers to maintain a minimum cash balance (Brown 2012). For demonstration phase projects (TRLs 7–8), repayment requirements and covenants can potentially increase cash flow burdens, leading to liquidity issues. Having these debt service obligations in uncertain market conditions could be yet another burden facing innovative technologies that already face a host of other challenges. Related to this, projects supported by LPO loans and loan guarantees typically have a long payback period, which may be a poor match for the ever-changing renewable energy market (Brown 2012). Innovations funded by other sources of private capital and federal programs could challenge the status quo of demonstration projects under LPO. Finally, with the large size requirement for selected projects and the LPO’s limited capacity to fund programs, the total impact may be smaller than desirable.
The lack of existing scholarship on the effectiveness of loan programs, as well as the scarcity of data on the net benefits of funded projects, makes it difficult to assess the magnitude of these challenges. In its annual portfolio status report, LPO publishes some data on avoided CO2 emissions and new jobs supported, but only on an aggregate basis (LPO 2022). Further empirical analysis would be necessary to fully understand the trade-offs of LPO’s programs.
3.2.1.3. ARPA model of research funding
The Advanced Research Projects Agency (ARPA) was first established by the US government in 1958 to improve the nation’s competitiveness in military and space-faring technologies. It later became the Defense Advanced Research Projects Agency (DARPA), shifting the focus of its projects and operations several times throughout its existence. The Advanced Research Projects Agency–Energy (ARPA-E), instituted in 2009, is an organization modeled after DARPA that seeks to fund innovations in the energy sector.
The ARPA model requires quantifiable goals and verifiable measures of progress. The intention of the program is to support high-risk, high-reward projects, with the idea that returns to innovation are considerably skew-distributed, with a few big successes that justify the support of many different projects (Scherer and Harhoff 2000). Additionally, novel discoveries or inventions may be discarded by experts in the field as a result of bounded rationality (Boudreau et al. 2016). This is addressed in the ARPA framework by accepting reviewer disagreement, as this implies uncertainty, which in turn may imply novelty (Azoulay et al. 2019). In ARPA-E, projects are often selected for funding even when they fall below the peer-review cutoff line. More generally, the ARPA model tackles this bias through individual discretion and selection of uncertain projects.
In terms of process, ARPA-E follows the strategy of applying organizational flexibility, with a considerable degree of discretion given to individual directors of programs as well as researchers. Within this design, project managers are given individual responsibility, often constrained only by specific technical targets (Azoulay et al. 2019). Moreover, research teams often comprise individuals from diverse backgrounds to stimulate synergies that would otherwise not form by themselves. The ARPA model also provides considerable flexibility for contracting, including the use of special types of agreements, such as with tailored terms regarding intellectual property. ARPA-E has been successful in developing nonincremental innovations, such as through its Grid-Scale Rampable Intermittent Dispatchable Storage (GRIDS ) program. The technical goal of $100 per kilowatt-hour was made a funding prerequisite, and the attainment of this target has become a fundamental step change, with the project benchmark becoming the new industry benchmark (Azoulay et al. 2019).
Transferring innovations to large-scale deployment in markets and facilitating the wider diffusion of relevant new technologies have been significant issues for the ARPA model in practice. This is especially the case when the innovation is not subject to public procurement or some other demand guarantee. ARPA-E, for instance, seeks to address this issue with its tech-to-market team but has been rather unsuccessful (Azoulay et al. 2019). Thus, while the ARPA model has seen major success in delivering innovations with its use of R&D on the supply-push side, it has had less success in addressing the required demand-pull factors needed for widespread technology adoption.
3.2.2. Demand-pull policies
In contrast to supply-push policies, which have traditionally been used by government in R&D, demand-pull policies, seeking to increase the demand for innovations, have been employed less frequently. We review the literature on the wide array of demand-pull policies in the following subsections.
3.2.2.1. Inducement prizes
Prizes are a demand-pull policy that were popular in the early days of innovation policy and have received increased interest in recent years. Inducement prizes are awards for achieving a specified ex ante goal, with the payout of the reward conditional on the winner achieving the goal. This ex ante specification distinguishes these prizes from awards that are paid out ex post for prior achievements. Inducement prizes may also encompass related forms of incentives that could be described as prizes, such as patent buyouts (Newell and Wilson 2005; Davis and Davis 2004) or advance market commitments (AMCs) (Williams 2012). Prizes can include payments of cash or incentives in different forms, such as fast-track regulatory approval in the case of neglected diseases in the United States (Kremer and Williams 2010). This “priority review voucher” was first proposed by Ridley et al. (2006).
The literature suggests that prizes are more effective than supply-push approaches at inducing nonincremental innovation when the desired goal is sufficiently well defined, but there is need for an inventive step to achieve the goal. Thus prizes seem to fit best for early TRL stages, notwithstanding that the highest-profile prize (the X-Prize for private space missions) is very late-stage TRL.
Good design of the prize contest is essential. In particular, prize design should feature technical specifications that are not too vague but not overly specific, with a goal that is relevant but also achievable and that can clearly lead to ultimate commercialization. A particularly difficult aspect of design is the size of the prize needed to stimulate the necessary supply response and to be consistent with the social benefits that could result from the desired innovation.
Good process is essential, too, such as certainty to participants about the conditions for winning, including transparent governance and predictable rules. Publicity about the prize is important as well, as having a larger and more diverse set of participants can increase the likelihood of success. Another promising option is to have multiple prizes or prizes for different stages of the innovation development process. The latter can be considered an example of milestone payments, discussed in Section 3.2.2.4.
This highlights a key disadvantage of prizes: they are paid out only when the innovation is complete, thus forcing innovators to raise capital in the hope that they will win the prize. However, prizes lower the risk to lenders or grantees relative to situations without a clearly defined payout at the end. And entering a prize competition may be viewed by the financial markets, shareholders, and others as a nonmonetary benefit, such as from the publicity associated with the prize and the learning and esprit de corps from mounting the effort to win (Murray et al. 2012).
At a 2020 NAS workshop on prizes, Hoyt Battey of DOE indicated that “prizes are well-suited when a desired outcome is cooperation and sharing of information among contest competitors,” noting that “DOE has found that when they choose to use a prize, outcomes have been better than what they would have achieved through a traditional grant.” The workshop also promoted prizes to help build a community of practice. To garner a large pool of contestants, a suggestion was made to include the offer of some compensation to losers in the competition announcement (NAS 2020).
3.2.2.2. Public procurement
Government procurement programs are another way of pulling green products into the market. Because purchases by federal and state governments represent a significant part of overall material demand in the economy, public procurement can play a vital role in shaping and creating markets for green products. This role requires the government to set new or follow existing standards for what a green product is and put in place a decision system for identifying winners among diverse bidders.
While green procurement is not usually thought of as stimulating nonincremental innovations, it may induce innovation, as measured by patent activity (Krupnick 2020). In contrast to traditional inducement prizes, however, the reward lies in the public demand for a certain product and may not be conditional on an innovative step underlying the procured product. In an early exploration of the influence of public procurement on innovation, Geroski (1990) finds that public procurement may act as an innovation policy tool under certain conditions and in certain cases, such as national defense. However, it may be rendered ineffective if winners are wrongly picked and national champions declared, which hampers competition. It can also go wrong if ambition is too high and bidders cannot meet the requirements for clean projects.
The current emphasis in the federal government is on green infrastructure projects, such as roads, bridges, and buildings, where green refers to the use of low-carbon-intensity cement and steel, for instance. This has external benefits to other governments and even the private sector through standard setting (Krupnick 2020) and the creation of rules about how low costs and other traditional attributes of a desirable project are to be balanced against low carbon intensity, which can be expected to raise project costs (Hasanbeigi et al. 2019).
Ultimately, the idea, beyond meeting immediate needs for roads, bridges, and buildings, is to stimulate clean product markets. However, one must watch for the effect of large procurements crowding out the development of private markets if the demand is too large (Krupnick 2020).
3.2.2.3. Advance market commitments
Advance market commitments (AMCs; sometimes called advance purchase commitments, or APCs) are a form of demand guarantee for yet-to-be-developed technologies. Sivaram et al. (2021) report that AMCs have shown notable success in the development of vaccines and are optimistic that these could also work for low-carbon technologies for industry. AMCs in this space would guarantee the sale of a given amount of carbon reductions or low-carbon-intensity products at an agreed-upon price. Those committing to future purchases are not limited to governments. Indeed, major AMCs have been underwritten in several cases by private sector companies seeking CO2 reduction credits toward their net-zero goals.
AMCs are related to inducement prizes in that they promise a reward for successfully developing a desired technology. In contrast to prizes, however, they represent a binding legal contract with a specific procurer of the technology, and the reward comes in the form of a demand guarantee, which can be from the government. This means that the reward payments are conditional on the actual use of the product to be developed and are thus subject to a “market test” (Kremer and Williams 2010). In a sense, advance market commitments by the government can be thought of as a form of government procurement that includes a commitment to purchase a specific product before the technology is fully commercialized.
First proposed by Kremer and Glennerster (2004), AMCs have mainly been applied in the pharmaceutical sector for the development of new drugs and vaccines in the context of neglected diseases. Before a product is developed or licensed, a number of sponsors underwrite a guaranteed price for a certain number of predetermined purchases (Kremer and Williams 2010). These purchases take place only when the technical requirements of the innovation are met. Additionally, under the provisions of the AMC agreement, sponsors can agree to guarantee top-up payments on realized demand (e.g., from a poor country purchasing the drug), while in turn, developers agree on a long-run price cap on the product or, alternatively, the licensing of their intellectual property to other manufacturers (Kremer and Williams 2010). AMCs showed spectacular success as a component of Operation Warp Speed to develop and scale up the production of vaccines for COVID-19.
Kremer and Williams (2010) suggest that AMCs can extend prizes such as those awarded by the X-Prize Foundation by conditioning the rewards on a market test. The types of market tests applied in AMCs could then inform more complex reward mechanisms, such as a medical prize fund.
AMCs are not a substitute for broad market development, as winning companies are picked with varying degrees of a market test. Also, an AMC recipient can fail to deliver. The success of AMCs in the pharmaceutical sector may not carry over when applied to greenhouse gas abatement. Capital costs are significantly higher in steel and cement, whereas new pharmaceutical products have large market potential. AMCs may thus be more costly overall in the materials sector than they are for vaccines.
Optimal AMC design also depends on the technological distance of the desired innovation. Closer targets may be more feasible, but distant targets could be more efficient. AMCs must also be appropriately sized to have an impact on decisionmaking. An AMC of a few million dollars is not likely to generate a significant change in behavior if the total capital investment in a new plant is $1 billion, as can be the case in the industrial sector.
3.2.2.4. Milestone payments
Providing milestone payments to innovators for the successful completion of innovative steps in the product development process can also support reaching certain innovation targets. Nemet et al. (2018) note that such payments can support learning from demonstration projects, as they base funding on created knowledge. Milestone payments can make R&D contracts more efficient by dealing with a number of problems in funding. these payments can be used to prevent asymmetric information between innovators and investors (private or public), as one party having more information than another, regarding the value of the project or the probability of success, may lead to underinvestment (Bhattacharya 2015). The knowledge created may also decrease risk aversion on the part of investors, increasing investment. The precommitment aspects of milestone payments can mitigate holdup, where one party may invest suboptimally because of concerns that it may not be able to fully appropriate the returns on its investment. Finally, by reducing the exposure to loss, milestone payments can reduce moral hazard, where one party is overly incentivized to take risks as it does not fully bear the downside consequences.
Milestone payments can be incorporated into different types of innovation incentives. For example, one could argue that the OCED’s Hydrogen Hubs Program is an example of a milestone-based approach, as it features four phases of funding, each based in part on performance in the previous phase. Milestone payments are also used in private R&D partnership contracts and in projects of ARPA-type agencies (Azoulay et al. 2019). Such payments have been successfully applied in aerospace technology and can complement other policies in early-stage innovation.
3.2.2.5. Product and carbon intensity standards
Following Swann (2000) and Blind (2016), standards can be seen as a type of infrastructure for innovation. They enable procurement programs that, for example, need carbon intensity benchmarks for deciding what is green steel and cement, and purchasers of green materials need to know whether they can trust these materials to perform adequately as an input to products (such as vehicles) and projects (such as building a bridge). Given that there may be trade-offs between various performance characteristics for steel and cement and the embodied carbon intensity, accepted standards for performance are essential for green product markets to develop.
Standard setting may have positive or negative impacts on innovation. Standards contribute to create additional opportunities for users, help reduce transaction costs, and facilitate trade of complex products, and they can form the basis of subsequent innovations. They naturally limit the options for new technologies to be realized, however. This can help in the development of critical mass in those technologies, which is a traditional concern of demand-pull policy in innovation. Moreover, by increasing credibility in the technologies, standards can attract further investments and spur the development of complementary technologies.
For established technologies, standards can help bring about economies of scale. The scaling up of technologies is an important component of overcoming the valley of death. Also, as Blind (2016) argues, standards may enable firms to generate the necessary profits to reappropriate some of their investments in innovation. In this regard, standards act as an effective leveraging and diffusion mechanism for patent rights. Finally, standardization reduces the risk of new products, which increases users’ trust in innovative technologies.
Standards also have some potential challenges and downsides. The main challenge is to set standards for the appropriate product classes. It would make no sense to set the same intensity standard for all steel products, for instance, since their carbon footprints are so heterogeneous. The Buy Clean California Act, for example, sets intensity standards for four steel products, from 0.9 t CO2e per metric ton for concrete reinforcing steel to 1.7 t CO2e for structural steel. Buy Clean California Act of 2017, Public Contract Code § 3500–3505, https://www.dgs.ca.gov/PD/Resources/Page-Content/Procurement-Division-Resources-List-Folder/Buy-Clean-California-Act Some other downsides are mentioned by Blind (2016) and Swann (2000), mainly pertaining to the potential adverse effects on market concentration. Market concentration and monopolistic market structures tend to stifle innovation by reducing the pressure to innovate. Specifically, standards may be dominated by single companies (e.g., if they hold the patents necessary for their implementation), leading to a concentrated market. Standards set at a high level of ambition might lead to prohibitively high implementation costs, which can deter new entrants and thereby reduce competition.
Even without intellectual property restrictions, standards have the potential to lock in outdated technologies (Blind 2016). Especially radical innovations are sometimes delayed significantly by the presence of standards. When placed appropriately, standards can help avoid lock-in on old technologies by ensuring compatibility between old and new technologies. However, standards run the risk of selecting technologies prematurely. Blind (2016) notes that these factors can mostly be mitigated by open and transparent standardization processes. More frequent updating of standards can help mitigate these lock-in problems as well. The design of the procurement program can also mitigate the problems with standards. One idea, suggested by Krupnick ( 2020), is to create multiple standards of ever more “greenness” and provide project scoring systems that award more points for meeting tighter standards in the bidding process.
Blind (2016) notes that implementing a new standard has a direct innovation effect on companies as potential suppliers. This then may have positive spillover effects into the private sector. Moreover, standards reduce the risks related to costs, health, the environment, and safety for the public procurer and consequently create leeway for the procurement of products and services with innovative characteristics. However, the implementation of technical standards in tenders requires a deep understanding of the technological environment and the market landscape in which the procurer is operating. Ghosh (2005) points out that the combination of patents, standards, and public procurement can lead to a triple dividend for dominant market participants, creating considerable market inefficiencies.
3.2.2.6. Carbon contracts for difference
A contract for difference (CfD) is a form of financial derivative that exchanges a fixed return for a floating return, in a manner similar to a commodity swap. In electricity markets, for example, a CfD would pay the difference between the wholesale price of electricity and a predetermined strike price. The price could be set either administratively or through an auction. This instrument has been used by governments to subsidize energy investment by removing price risk. The use of CfDs by state governments to subsidize generation was ruled to be in violation of the Federal Power Act in Hughes v. Talen. A number of variations on this instrument have arisen in recent years. In one variant discussed by Bollerhey et al. (2022), an entity is created that procures a clean commodity such as hydrogen via long-term contracts. The entity then sells the commodity to off-takers on a short-term basis through a competitive bidding process. The government will cover any losses due to price differences in the contracts. In this way, the entity provides both price and duration certainty to the producers, reducing their risk. The competitive nature of the procurement and sales should, to some extent, reduce the risk to the government to cover the entity’s losses and bridge the gap in prices between the supply and demand sides over time.
Another variation on the CfD gaining increased attention is the carbon contract for difference (CCfD). Unlike the example of Bollerhey et al. (2022), this policy’s purpose is to counter the innovation-depressing effects of the uncertainty and volatility of an allowance price in a cap-and-trade system (or other carbon price policy) rather than the price of a physical commodity. With CCfDs, if the carbon price is below a government-set strike price, the government will pay the difference between the strike price and the actual price of carbon multiplied by the abatement achieved by the contractee. In some versions of a CCfD, the contractee must pay the government if the carbon price rises above the strike price.
By derisking the emissions price, CCfDs can boost investor confidence and help commercialize new low-carbon technologies. This instrument may not make much sense in the United States because of a lack of general carbon pricing outside of California and the Northeast. With appropriate design, CCfDs can be highly cost-effective in reducing risk. Proper design must set the right strike price and select eligible projects through a tender process.
3.2.2.7 Additional demand-pull features
Many demand-pull tools involve a price being set: the prize amount, the strike price of a CfD or CCfD, and the price paid for CO2 reductions in an AMC, for example. Other tools, such as green procurement, are inherently competitions that set prices—in this case, prices on projects (e.g., building a road) rather than prices of products (e.g., steel) or processes.
However, price setting can be tricky when market forces are not present. In such cases, one option is to use an auction among competitors. When sellers compete to offer the most attractive price for their supply decision, this is called a reverse auction and would apply to some of the demand-pull policies. For instance, competitors for a prize could include in their application the prize they need to supply the performance terms. Similarly, an AMC could be structured to get the applicants to state the price they need to supply the requisite CO2 reductions. In these cases, the demander (or buyer), whether government or private sector entity, could decide to take the lowest bid or consider other factors in deciding which bid or bids to accept. The buyer, if terms allow, might also use these revealed prices to set a prize amount or strike price with more certainty than without a price discovery process.
3.2.3. Regulations and Tax Credits
Supply-push and demand-pull policies are those focused directly on innovation and often on specific technologies or systems in need of progress. These approaches are sometimes embedded in a complex web of alternative activities driving innovation directly or indirectly.
The first of these is regulation. We do not consider the advantages and disadvantages of all types of regulations here, but in general, for efficiency, taxes, tradable permits, and to a lesser degree tradable performance standards are preferred because they let the regulated entity decide the most cost-effective approach to reduce emissions. While these incentives can lead to innovation, they may instead drive the deployment of extant emissions reduction technologies. And to the extent that standards must be set and met, they discourage innovation efforts greener than the standard.
A form of environmental regulation is mandates, such as renewable portfolio standards, which we use here to illustrate the advantages and disadvantages of this form of regulation to innovation. These standards mandate that electric utilities demonstrate that a certain percentage of their generation is from renewable sources, such as wind and solar power. These standards exist in many states but not at the national level. Unquestionably, these standards (along with supply-push funding from DOE) have pushed innovation in renewable energy technology to bring costs down to a remarkable degree—both from economies of scale in their production and from stimulating technological innovation. However, they encourage utilities to just meet the standard but not go beyond it. They also are not particularly applicable to lower-TRL technologies unless the standard is designed with long lead times in required compliance.
The flip side of regulations is subsidies, which get actors to do something by making it worth their while rather than by requiring compliance. The spate of tax credits in the Inflation Reduction Act (for clean hydrogen and carbon capture, for instance) fall into this category. Economists are generally suspicious of subsidies because they can encourage bad behavior to be eligible for the subsidies and they build strong constituencies for keeping them going. They are often open-ended (as the new credits are) and lack a strong accountability system (except IRS audits), and they reduce government revenue or raise government expenses. Maybe worst of all, when particular technologies are targeted, they pick winners rather than let the market decide, as some other forms of regulation do. That is, subsidy programs tend to be sector, fuel, or technology specific when a better approach is to regulate through performance standards or taxes and tradable permits that directly target emissions and let the market decide which solutions make economic sense.
3.2.4. Summary of Tools
In our review of tools to deliver greenhouse gas mitigation innovations, we have examined a variety of attributes for judging the applicability of a tool: the scale and scope desired, the readiness level of the technologies involved, the number of competitors, the size of the budget, and many others. It would be desirable to have a checklist whereby tools could be chosen based on their performance with respect to the attributes of the particular case in need of a tool, but alas, with so many attributes and relatively few tools, such a tidy approach is not feasible in general. Nonetheless, our analysis has highlighted some relations. For example, prizes seem to work best with earlier-stage technologies and a number of competitors. With an advance market commitment, it is clear that the size must be sufficient to have a material impact on the economics of the producers. In Section 5, where we consider applications specific to the steel and cement sectors, we match tools to specific situations where innovation is needed.
4. Developments in Government Funding for Innovation
In this section, we discuss some developments in both supply-push and demand-pull government policies directed at the industrial sector, as well as tax credits that apply to any sector. A number of these demand-pull policies are already employed in the energy sector. Three major pieces of legislation passed in 2021–22 and an important executive order on green procurement gave these efforts a huge boost. In addition to reviewing the impacts of the three laws, this section provides an overview of ongoing federal government activity in the use of prizes and in green procurement.
4.1. Relevant Legislation
4.1.1. Infrastructure Investment and Jobs Act
In late 2021, the Infrastructure Investment and Jobs Act (IIJA), also known as the Bipartisan Infrastructure Law, appropriated $1 trillion of public investment to upgrade roads, bridges, power grids, and other infrastructure all over the country during 2022–26. Of most relevance here, the package allocates $31 billion for RD&D in green technologies, mostly to be administered as competitive grants by DOE. The funds cover RD&D in clean hydrogen and carbon capture, advanced batteries, advanced nuclear, and direct air capture technologies.
A key piece of the legislation focuses on CCUS technologies. The IIJA expands several DOE programs, with $310 million targeted to the Carbon Utilization Program to include the development of standards and certifications to support commercialization of carbon oxide products. Along with the standardization of carbon oxide products, this program awards grants to local authorities to use or procure products derived from the capture of carbon oxides. By focusing on the commercialization of carbon capture outputs, this piece of the legislation can be understood as a demand-pull instrument. The bill also expands the Carbon Capture Technology Program to include pipeline infrastructure, with an additional $100 million over the next five years. This is to be administered by the National Energy Technology Laboratory (NETL), which awards funding to selected RD&D projects. These funds are not for RD&D itself but would facilitate market growth.
Other programs implemented or expanded by the IIJA include the Advanced Energy Manufacturing and Recycling Grant Program ($750 million), the Carbon Dioxide Transportation Finance and Innovation Program ($2.1 billion), and the Large-Scale Carbon Storage Commercialization Program ($2.5 billion). The IIJA also adds funding to the existing Carbon Capture Large-Scale Pilot Programs ($937 million) and Carbon Capture Demonstration Projects Program ($2.5 billion). Similarly, the Carbon Removal Program provides $3.5 billion for the development and demonstration of four regional direct air capture (DAC) hubs. The IIJA legislation also appropriates new funding for DAC Technology Prize Competitions (DOE, n.d.).
Funding for green hydrogen is another key part of the IIJA, with $9.5 billion for different programs in the sector. The bill expands the scope of DOE’s Hydrogen Research and Development Program, focusing on the demonstration and commercialization of clean hydrogen production, processing, delivery, and end-use application technologies. Additionally, while mandating the development of a national strategy and roadmap to facilitate a clean hydrogen economy, the bill establishes three new RD&D programs: the Regional Clean Hydrogen Hubs Program ($8 billion), the Clean Hydrogen Manufacturing and Recycling Program ($500 million), and a demonstration, commercialization, and deployment program to decrease cost of clean hydrogen production from electrolyzers ($1 billion). Eligible entities will receive grants on a competitive basis from DOE.
The IIJA allocates $10M to demonstration projects for energy storage of intermittent renewable electricity. While not directly relevant to industrial decarbonization, energy storage is important for complementing plans to make the grid as low-carbon as possible and therefore lower the carbon footprint of industries choosing to substitute electricity for fossil fuels in their processes or to meet heating needs. The bill provides funding to two other energy storage programs, the Energy Storage Demonstration Projects and Pilot Grant Program and the Long-Duration Demonstration Initiative and Joint Program, amounting to $355 million and $150 million, respectively.
The Advanced Reactor Demonstration Program receives $3 billion for the period 2022–26. Again, while not directly linked to industrial decarbonization, this program could help make the grid cleaner. Similarly, while battery development is not directly tied to industrial decarbonization, the IIJA supports the DOE’s ongoing Lithium-Ion Battery Recycling Prize with an additional $10 million to carry out Phase III. These programs are open to both private and public entities.
The IIJA also created the Office of Clean Energy Demonstrations (OCED), discussed in Section 2.2, to be in charge of the management of demonstration projects. OCED conducts project management and oversight of all the demonstration projects we have mentioned and more, representing $22 billion of investment in demonstration projects. In early 2023, OCED issued a request for information (RFI) on the use of demand-side mechanisms to support clean energy. This RFI focuses on many mechanisms discussed in this paper, including direct procurement, AMCs, and price floors (which it takes to include CfDs).
4.1.2. Inflation Reduction Act
Passed in the fall of 2022, the Inflation Reduction Act (IRA) created or modified many relevant programs for industrial decarbonization. Most important are two tax credit programs, 45Q and 45V, for promoting CCUS and clean hydrogen, respectively. The first program provides $85 per metric ton for captured and sequestered carbon oxides. This is a substantial increase from the maximum of $50 per metric ton available under previous iterations of this tax credit. The 45Q tax credit increases to $180 per metric ton for carbon dioxide sequestered from direct air capture. The 45V tax credit provides up to $3/kg H2 for hydrogen production with a life-cycle carbon intensity below 0.45 kg CO2e/kg H2, with lower amounts for more carbon-intensive production, falling to zero for life-cycle emissions above 4.0 kg CO2e/kg H2. These tax credits are explained in more detail in an RFF report by Krupnick and Bergman (2022).
The IRA makes a substantial investment in green procurement, discussed in Section 4.2. Numerous other tax credits in the bill are aimed at reducing emissions from electricity production; increasing the availability of components of clean energy technologies, including critical minerals; reducing the cost of electrification of demand in the residential, commercial, and transportation sectors; increasing the adoption of clean vehicles; and deploying clean fuels. With respect to the industrial sector, the IRA includes $5.812 billion for OCED for the Advanced Industrial Facilities Deployment Program and a revival of the 48C tax credit, with $10 billion for clean manufacturing. The IRA appropriates $11.7 billion to the LPO to support approximately $100 billion in new loans, as well as $5 billion for the new Energy Infrastructure Reinvestment Program in the LPO, with authority to provide loans up to $250 billion. This legislation also includes a large number of provisions not directly related to the industrial sector, such as funds for a Greenhouse Gas Reduction Fund (related to the green bank concept) and a fee on methane emissions.
4.1.3. CHIPS and Science Act
Domestic manufacturing of microchips and semiconductors is at the core of the CHIPS and Science Act passed during the summer of 2022, with an investment of $50 billion in the development of an American supply chain. In addition to the semiconductor funding in the bill, it appropriates $67 billion for DOE, with the majority going to the Office of Science, which will fund early TRL R&D of clean energy and advanced computing technologies (DOE 2022e).
This legislation also establishes a new nongovernmental foundation, the Foundation for Energy Security and Innovation (FESI), which will collaborate with DOE to reach goals such as advancing R&D to tackle climate change and protecting energy independence. FESI will be in charge of encouraging public-private partnerships between government, industry incumbents, start-ups, and other funding entities to foster collaboration in innovation ecosystems and bridge the valley of death (Duggan and Hart 2022).
4.2. Green Procurement Initiatives
Beginning in 2021, a number of administrative and legislative actions have combined to set up the federal government and, through the federal Highway Trust Fund (a $49 billion/year road and bridge funding support program), state governments to procure roads, bridges, and new building construction that use steel, cement, and other materials with a lower carbon footprint. In the FY2023 Omnibus Appropriations Bill, DOE was directed to start a program to purchase carbon dioxide from direct air capture. DOE has not yet announced how it will implement this directive, so we focus here on federal procurement of green construction materials.
In December 2021, the Biden administration issued Executive Order 14057 on Catalyzing Clean Energy Industries and Jobs through Federal Sustainability. This includes a Buy Clean Task Force to make recommendations on how to promote the use of low-carbon steel, cement, and other construction materials in federally funded projects. The General Services Administration (GSA) has already issued a set of new rules, which set limits on the embodied carbon of concrete procured for its construction projects.
The IRA appropriates over $2 billion to the Federal Building Fund for efforts that support the use of low-carbon materials in buildings and construction under GSA’s control. The legislation also appropriates $2 billion to the Federal Highway Administration to incentivize the use of low-carbon materials in construction projects. It appropriates $100 million to EPA to develop a labeling system for construction materials that have a substantially lower carbon footprint than the industry average to be used in these construction projects. These provisions amount to $4.5 billion to help boost the Federal Buy Clean Initiative. Another $3 billion is slated to go toward the purchase of electric vehicles and associated infrastructure (e.g., charging stations) by the US Postal Service.
Further, the IRA provides $250 million for EPA to develop environmental product declarations for whatever materials sectors and products the agency deems appropriate. Environmental Product Declarations (EPDs) are third-party verified documents specifying the emissions and other types of waste products and environmental results. An EPD includes information on the manufacturer, product characteristics, life-cycle assessment methodology used, material feedstock and chemical content, global warming potential, waste streams, and potential environmental certifications. However, an EPD contains no information related to compliance with any environmental standards. The federal government will likely develop standards for use in EPDs to comply with the Buy Clean Initiative.
EPDs can be used to compare environmental performance between similar products made by different manufacturers or using different technologies. Green building certifications and rating systems, such as LEED, often require EPDs during the evaluation process. EPDs are already widely used in the cement and concrete sector. The New Buildings Institute collected data from more than 36,000 US-based ready-mix concrete EPDs (NBI 2022). This large number can be explained by the fact that EPDs in the cement and concrete sector are product-based and hundreds of mixes are possible with various levels of each ingredient, especially to make concrete. By comparison, steel is a highly standardized product, so there are fewer product-level EPDs. In the same study, only 100 international EPDs for steel were collected, with some products widely underrepresented.
The Federal Buy Clean Initiative prioritizes the use of low-carbon construction materials for federally funded projects and requires EPDs to measure the embodied carbon of the procured materials. GSA has set limits for each class of concrete at 20 percent lower than the industry limit set at the 75th percentile of all embodied carbon values by NBI (2022). The Buy Clean California Act also defines embodied carbon limits for structural steel using the industry average for each type of steel product.
4.3. Prizes
DOE first received the authority to issue prizes under the Energy Policy Act of 2005. We have found reference to an efficient-lighting prize competition as far back as 2008 (Brennan et al. 2012). Prizes were given a big boost throughout the federal government in 2011 when the America COMPETES Reauthorization Act became law, authorizing a broader use of prize competitions than under existing authorities. This authority was then modified under the American Innovation and Competitiveness Act of 2017. Every other year, under the COMPETES Reauthorization Act, the Office of Science and Technology Policy in the Executive Office of the President issues a report on the use of prizes throughout the federal government. For the most recent report as of this writing, see https://www.whitehouse.gov/ostp/news-updates/2022/05/04/white-house-office-of-science-technology-policy-releasesfiscal-year-2019-20-federal-prize-and-citizen-science-authority-report/.
A US government website lists all federal prize competitions and serves as an information hub for federal prize managers and others; DOE prizes are listed on this site, but DOE also has its own prize websites. The federal prize website is https://www.challenge.gov. One of DOE’s prize websites is https://americanmadechallenges.org, run by the National Renewable Energy Laboratory. As of February 2023, nine open prize competitions were listed. Competitions cover such things as innovation in energy storage, wind energy, developing high conductivity cables, digitizing electric utilities, and advanced building lighting systems. Together, over $170 million in prize money is offered. An additional 16 prizes are in progress and 20 competitions are closed. The numbers of competitions and funds available pale in comparison to policies using supply-push tools. However, most challenges are tightly focused and for low-TRL technologies, which do not necessarily require expensive efforts.
In considering prize processes and designs, DOE’s EERE issued a prize guidance document that details all the necessary design steps (EERE 2019). The guidelines note that prizes are especially suited to, among other things, increasing the number and diversity of innovators involved, encouraging risk-taking while having transparent and predictable rules, and reaching innovation goals without picking winners. These characteristics are also highlighted in the academic literature for prizes.
The prize goals, reward size, winning criteria, and other rules are defined at the very beginning of the EERE prize process. They are included in a comprehensive prize rule document, which serves as a reference for the participants. In addition, to address potential uncertainties about winning conditions, a mandatory webinar and public Q&A platform must be put in place after publication of the rules. Prize managers are encouraged to consult different experts and other EERE staff who have successfully developed prize competitions to inform their decisionmaking. Wider consultations, through RFIs and workshops, should be held when selecting prize topics. This helps define the goals and other characteristics of the competition.
EERE guidance promotes the use of different prize designs to achieve different missions and goals. The guidelines establish that precise technical goals and the size of the reward should be set according to the issues the prize program is looking to address. Prizes promoted by EERE do not necessarily require specifying a particular technological solution, which prevents favoring one pathway over another (Murray et al. 2012) and enables nonincremental innovation.
Flexibility provided by the staging of prizes with down-selection can help ensure that rewards go to the best innovators while attracting a large pool of applicants that are new to government funding (DOE personnel, interview by authors, February 2022). The overall flexibility in design possibilities in the EERE guidance enables EERE staff to establish goals, reward sizes, and performance criteria that are relevant to each prize competition.
EERE does not provide particular guidance on how to ensure market viability after a prize competition. However, the literature highlights that these programs should send a positive signal to financial markets and potential investors related to the efforts the awardee made to win. Overall, EERE guidance seems to address the elements that make prize programs successful (e.g., relevant and achievable goals, staging, certainty, and transparency regarding rules).
To examine the key design features of these prizes followed by DOE, we provide the example of a $4.5 million prize issued by the Advanced Manufacturing Office (AMO) in 2022, dubbed CABLE, for conductivity-enhanced materials for applications in moving electricity and heat with lower resistance. This is a three-stage process, with winners at each stage and up to four winners at the end. AMO explains, “Unlike Stage 1, in which competitor’s [sic] scores were determined by submission materials—especially the Technical Narrative—in Stage 2, 50% of each competitor’s score will be determined directly by electrical conductivity testing (relative to related inputs in Appendix C) and 50% will be determined by reviewers based on the Technical Narrative and the other submission materials” (DOE 2022a, 6).
The rules contain many specific technical details concerning standards to beat, and the prize includes three different sets of competitions based on existing material conductivity. Here is one:
Contest 1: Beat Copper! The first contest asks competitors to produce a material with a test-sample conductivity that significantly exceeds that of commercial electrolytic copper (set in Appendix A as 58.6 MS/m [101 % IACS]). The material with the highest tested conductivity will get the maximum number of testing points. Other requirements include that the material contains no silver and that the submission includes a credible description for eventual (10-years-post-commercialization) economic competitiveness with electrolytic copper as well as parity or improvement on all other relevant copper performance requirements (e.g., mechanical and anticorrosion properties) (DOE 2022a, 9).
We note the requirement to include an analysis or credible description of how this material can become economic against its nearest competitive material. A point-based scoring system is also provided and, as is typical now, the proposal must include a diversity, equity, and inclusion plan.
This example of a prize implementation seems to follow the EERE guidelines. The competition is staged, with increasingly large prizes awarded to a decreasing number of winners. The prize developers made use of the design flexibility allowed in the guidelines to allow a mix of technical requirements (in the first stage) and performance criteria (in the second stage) adapted to this specific competition. Goals also address the prize’s overall mission, which is to develop “affordable conductivity-enhanced materials and applications” (DOE 2022a, 5). An RFI was released to collect feedback on testing requirements and performance criteria as advised in the EERE guidance. Finally, clear and transparent rules and winning criteria were made available to applicants in advance.
4.4. The ARPA Model
In February 2021, the Biden-Harris administration proposed creating the Advanced Research Projects Agency–Climate (ARPA-C), based on DARPA and ARPA-E, with the goal of developing technologies to aid the decarbonization of the economy (White House 2021). The ARPA model not only is gaining ground in new technological areas but also has served as a blueprint for similar organizations in other countries, such as the UK’s Advanced Research and Invention Agency.
5. Applications to Iron and Steel and Cement Sectors, and Crosscutting Technologies
In this section, we evaluate the opportunities to use demand-pull policies to decarbonize the iron and steel and the cement and concrete sectors. We separately address technologies that cut across these two sectors and others, including CCUS and process heat. For each sector, we describe the industry, the technologies currently used in production, and new technologies that can enable decarbonization. Although, in the short- to mid-term, technologies providing marginal GHG reductions can be important, we will focus on innovations that can bring about the deep decarbonization in these sectors that is necessary to achieve net-zero goals. We next discuss the funding situation for these technologies and the potential applicability of demand-pull mechanisms in each sector. Finally, we look at two crosscutting technologies that have applications in both sectors.
5.1. Opportunities for Application to Iron and Steel
Ironmaking is the process of removing the oxygen from iron ore, which is generally made up of iron oxides, molecules containing iron and oxygen, leaving elemental iron. To create steel, the carbon content of the iron must be adjusted, and other elements are potentially added to create different alloys. Since iron ore generally contains a number of other elements, purification of the iron must occur at some point in the process. The emissions from steelmaking generally come from two sources: removing the oxygen from the iron ore, called reduction, and generating the heat needed to melt the iron and, if necessary, remove any excess carbon.
Iron and steel manufacturing is one of the largest sources of industrial emissions in the world, representing over 25 percent of global industrial emissions and 7 percent of total worldwide emissions. The sector produces 1,808 Mt of crude steel annually, with China accounting for over half of the production. The US steel industry is the fourth-largest producer globally, with 87 Mt of crude steel produced in 2018, and accounts for 4 percent of US CO2 emissions and 24 percent of industry-related emissions (DOE RFI). Because of the large amount of capital investment needed and the relatively high TRL of at least one decarbonization technology, traditional deployment funding is likely the best method of reducing emissions in this area, while continuing to fund the basic R&D needed for the low-TRL technologies.
5.1.1. Industry Overview
Iron and steel plants are large undertakings, with integrated steel plants usually costing about $1 billion to $4 billion (OneMonroe 2019) and often taking several years to build. This paper considers only the manufacture of iron and steel from iron ore. A large industry exists that uses scrap metal as a feedstock, usually in “mini-mills” that use electric arc furnace (EAF) technology. Since this process does not include the reduction process that removes the oxygen from the iron ore and uses EAFs, it has relatively low direct emissions. Indeed, increasing the amount of scrap through increasing the efficiency of the scrap market is often cited as a pathway (albeit limited) toward decarbonizing this sector (Fan and Friedmann 2021).
In 2022, the US steel industry included 11 active integrated steel plants producing pig iron and raw steel, owned by three companies. The EAF segment was less concentrated, with 101 active mini-mills producing raw steel operated by 50 companies. There were also three sponge iron production plants delivering their products mainly to mini-mills. This means that, relative to the rest of the world, the US iron and steel industry was already relatively clean, as only about 30 percent of US steel was produced in integrated steel plants, while 70 percent came from cleaner EAFs (USGS 2023).These proportions are reversed when looking at the global steel production (IEA 2020b).
Most of the steel in the United States is produced in the Northeast, although Indiana is the top-producing state, accounting for 26 percent of national production in 2022 (USGS 2023). The United States is highly trade exposed, as it is a net importer of steel products (Broekhoff et al. 2021). The main sources are Canada, Brazil, Mexico, and South Korea, cumulatively accounting for 59 percent of imports (USGS 2023).
5.1.2. Technology Background
5.1.2.1. BF-BOF
The most common and most emitting means of producing steel globally uses a blast furnace–basic oxygen furnace (BF-BOF) process to generate steel from iron ore and coke derived from coal. Iron ore is preprocessed through sintering and pelleting plants, while the coke is processed in a coke oven. Both materials are then heated in a blast furnace, usually using fossil fuel such as coal or natural gas for heat. The coke then reduces the oxygen from the iron ore through a variety of chemical reactions that occur at different temperatures, often forming carbon monoxide from oxygen and carbon dioxide, with the carbon monoxide acting as a reducing agent. This first step is the most energy-intensive and produces the majority of carbon emissions (both carbon monoxide and carbon dioxide). Since not all the coke is used up in the reduction process, the resulting iron has too much carbon. Carbon is removed by blowing oxygen over the iron in a basic oxygen furnace to achieve the appropriate levels for steel. The basic oxygen furnace also melts the iron, and limestone is added to remove impurities. The resulting hot steel is then ready for casting and rolling (Rissman et al. 2020).
In the United States, about one-quarter of steel is produced using this method (Hasanbeigi and Springer 2019). However, this process is responsible for two-thirds of the sector’s GHG emissions. Because of the multiple sources of CO2 emissions, integrated steel plants using the BF-BOF process are especially hard to decarbonize (Fan and Friedmann 2021). Capturing CO2 emissions is likely the best solution to retrofit existing integrated steel plants (see Section 5.3.1 for a discussion of carbon capture).
Other solutions to reduce emissions from BF-BOF steel production rely on performance improvements for the different parts of the process and on material efficiency (IEA 2020b). For instance, recycling flue gas from the various exhausts in the blast furnace is a relatively easy retrofit that increases energy efficiency by lowering the coke requirements for the process (Ryan et al. 2020). However, emissions reduction from these efficiency improvements do not come close to achieving complete decarbonization.
5.1.2.2. DRI-EAF
A second means of producing steel makes use of EAFs, which can melt recycled metallic scrap (called secondary steelmaking) while using only electricity as an energy source. Thus the direct emissions for EAF steelmaking are very low, with additional minor emissions from other parts of the steelmaking process. Instead of scrap iron, direct reduced iron (DRI) produced from iron ore can also be used in EAFs in a process called DRI-EAF. Direct reduction of iron is the process of reducing iron ore using syngas, a mixture of hydrogen and carbon monoxide usually made from fossil fuels. The oxygen from the iron ore binds with the hydrogen and carbon monoxide, creating water, carbon dioxide, and elemental iron. The DRI is then formed into briquettes that can be fed into EAFs to produce crude steel, which can be transformed into finished steel products through casting and rolling (Rissman et al. 2020).
In the United States, making steel using the EAF route is less carbon-intensive than the BF-BOF route. Producing steel through the EAF route emits 0.58 t CO2 per metric ton of crude steel, while the global average is close to 1.4 t CO2 per metric ton, when accounting for scope 1 and 2 CO2 emissions (Hasanbeigi 2022). However, secondary steelmaking—that is, making steel from scrap using EAFs—is not sufficient to decarbonize the sector. Even if more than 80 percent of all steel products in the United States are recycled at the end of their life (AISC 2017), scrap steel availability can be an issue for secondary steelmaking. Moreover, secondary steel can be of a lower quality than primary steel and may not be suited for some uses (EIA 2022).
As with BF-BOF steelmaking, emissions can be reduced from DRI steelmaking using carbon capture, as discussed in Section 5.3.1. With DRI, there is also the opportunity to replace the syngas used as a reducing agent with pure hydrogen. Eliminating the carbon monoxide means that only water remains as a process emission from the reduction of the iron ore (Rissman et al. 2020). However, unlike the reduction using carbon monoxide, which generates energy, reduction using hydrogen requires more energy (Spreitzer and Schenk 2019).
Hydrogen DRI prototypes at scale are planned or in construction in several different European countries. For example, the HYBRIT project from the Swedish steelmaker SSAB already has an operational pilot producing carbon-free steel with hydrogen instead of natural gas (Krüger et al. 2020). Following the success of the pilot, an industrial-scale demonstration plant is planned for 2026.
For certain preexisting DRI plants, it is possible to substitute hydrogen for up to 30 percent of natural gas (IEA 2020b). However, higher blending of hydrogen in the reducing gas at existing DRI plants requires changes to the facility equipment to generate the additional heat needed because of the energy consumption of the hydrogen reduction process (Hornby and Brooks 2021).
One potential hurdle with DRI-EAF steelmaking is the limited amount of high-quality iron ore available globally. Current DRI-EAF pathways require the use of pellets with an iron content of at least 67 percent, which is higher than for BF-BOF steelmaking (Nicholas and Basirat 2022). Technologies are under development to enable the use of lower-grade iron ore with hydrogen direct reduction methods. None of these technologies have been demonstrated at an operational scale.
5.1.2.3. Electrolysis
Electrolysis steelmaking, which is still at the small pilot level (TRL 5) of development, uses electricity to produce liquid elemental iron from iron ore. In this process, iron ore is placed in an electrolyte bath where an electric current releases the oxygen contained in the iron ore, leaving a high-purity liquid metal (Wiencke et al. 2018). The output of this one-step process can then be sent directly to casting and rolling facilities without reheating.
A version of this reaction developed by Boston Metals, molten-oxide electrolysis (MOE), requires a high temperature (1600°C). The company is working on an industrial-scale pilot and plans to build an at-scale demonstration plant by 2024. Another electrolysis pathway is being explored by a European project focusing on low temperature electrolysis, with a small-scale pilot on the way (West 2020).
Electrolytic steelmaking cannot be retrofitted on existing plants and will require entirely new facilities. However, the cost of this technology will likely be directly competitive with existing steelmaking even without carbon pricing, thanks to the ability to use lower-grade iron ore (Nicholas and Basirat 2022). Overall, capital expenses associated with MOE are expected to be lower than those associated with the BF-BOF route.
5.1.2.4. Smelting reduction
Smelting reduction is an alternative ironmaking process that does not require the use of coking coal. Furthermore, it facilitates the use of fine iron ore rather than the pellets used in the traditional BF-BOF route, decreasing the need for preprocessing of ore. This process has a 30 percent lower carbon intensity than traditional steelmaking and produces a more concentrated CO2 waste stream facilitating carbon capture (Quader et al. 2016). Steel production through smelting reduction has already been demonstrated in a Dutch plant (Fleschen 2018), but carbon capture was not implemented. Smelting reduction plants require entirely new facilities to replace blast furnaces, which will lead to large capital costs. Without carbon capture, this process cannot eliminate all the carbon dioxide emissions from steelmaking.
5.1.3. Funding Roadmap
5.1.3.1. DOE
In 2022, DOE released an FOA focusing on industrial decarbonization, calling for both small, laboratory-scale projects and larger, pilot-scale technology demonstrations in an operational environment (OCED 2022b). This funding opportunity allocates up to $14 million for iron and steel, with $4 million for low-TRL (4–5) projects and $10 million for higher-TRL (6–7) projects, subject to cost-share rules that require higher private sector shares for higher-TRL projects.
Innovations in the steel industry (e.g., low-density steels, molten oxide electrolysis) have been funded by DOE mainly through multitopic funding opportunities under the Advanced Manufacturing Office (EERE 2018). The AMO FY2020 multitopic FOA funded seven projects related to innovative iron- and steelmaking processes for a total grant portfolio of $21 million (EERE 2021). ARPA-E is also interested in funding low-emissions steelmaking. It plans to release an FOA for selecting projects that will enable carbon-free steelmaking at a lower cost than existing natural gas–based DRI (ARPA-E 2021).
In 2022, OCED received $5.812 billion for the Advanced Industrial Facilities Deployment Program under the IRA. A notice of intent for this funding lists iron and steel as an area of interest (OCED 2022c). OCED is also set to announce a funding opportunity for $500 million appropriated under the IIJA for Industrial Emissions Demonstration projects, beginning in 2023.
5.1.3.2. Private sector efforts
While several steel companies and associations representing one-third of global primary steel production have set ambitious targets to reach net-zero emissions by 2050 or before (Vogl et al. 2021), the US steel industry has not yet adopted a whole-industry decarbonization target to meet US energy and climate goals. However, industry publications and interviews with US steel industry representatives indicate that the industry needs and expects federal support for key crosscutting decarbonization enablers, including cheap renewable electricity, clean hydrogen, and CCUS equipment adapted to the industry characteristics.
In addition to federal R&D grants for new technologies, the industry also supports the use of incentives such as the 45Q tax credit for carbon capture. Federal funding for pilot-scale projects to develop new clean technologies is also supported by industry. Green procurement represents a significant opportunity for steel companies, but they would like more transparency on setting carbon intensity thresholds. They also highlight the fact that other competing construction materials should be held to the same kind of carbon intensity requirements in procurement programs.
At the company level, Cleveland-Cliffs, one of the largest US steel producers, having acquired AK Steel corporation and most of ArcelorMittal’s US assets, has committed to reduce greenhouse gas emissions by 25 percent by 2030 compared with 2017 levels. It plans to attain this objective by developing the production of hot briquetted iron, which is a high-purity form of DRI made using natural gas that can be fed into blast furnaces and EAFs to decrease GHG emissions. The company will also invest in the development of carbon capture technologies for its blast furnace operations (Cleveland-Cliffs 2021). As of December 2022, Cleveland-Cliffs was nearing completion of a DOE-funded feasibility study to demonstrate ex-ante carbon capture applied to blast furnaces at its Burns Harbor plant, and was seeking additional funding from DOE, which they would match, to cover the cost of the experiment (Pete 2022). ArcelorMittal has a net-zero commitment by 2050 for its global activities, with a most stringent midterm goal for the European market. It sees the United States as a region for more incremental improvements in the short term, while more climate-friendly policies in the EU or Canada create opportunities to accelerate its decarbonization strategy (A. Morelato, CTO ArcelorMittal North America, interview with authors, 2022). Similarly, Nucor has set a 35 percent reduction target in 2030 compared with 2015 levels.
5.1.4. Applicability of Demand-Pull Mechanisms
Besides carbon capture, the main technologies to decarbonize steel production are DRI-EAF using hydrogen and electrolysis (see Section 5.1.2.2). Hydrogen DRI-EAF is already being demonstrated in the HYBRIT project, and the electrolysis technologies are in the pilot stage. Given that these high-TRL technologies exist and have the potential to almost completely decarbonize the sector, funding for demonstration projects is likely the most important funding mechanism. Advance market commitments and government procurement are demand-pull tools that could also help deployment, but given the multibillion-dollar cost of a single steel plant, large sums of money would have to be devoted to such mechanisms. There may be opportunities for funding technologies that enable the use of lower-purity iron, however.
5.1.4.1. Prizes
Prizes are particularly suitable for early-TRL innovation responding to a well-defined challenge. Prizes are generally awarded after a project delivers a prototype of a product or a process reaching a specific target. While the production of low- to zero-carbon steel would be a relatively straightforward goal for a prize, the technologies currently developed are at fairly high TRLs, making prize funding less appropriate. Prizes are considered efficient instruments for funding, since relatively small awards can encourage large private investments from innovators to win the competition. In contrast, a huge investment is needed to build a prototype, even at a small scale, in the iron and steel industry, with a steelmaking facility costing several hundred million to billions of dollars.
Finally, to fully realize the benefits of a prize, there must be competition among multiple innovators. However, the steel industry for primary production is highly concentrated in the United States, with only two firms owning all the integrated steelmaking facilities. These two incumbent firms also own two of the three US DRI plants. The secondary steel market is more competitive, with smaller EAF facilities under diverse ownership scattered across the United States.
5.1.4.2. Procurement and AMCs
Similarly to prizes, a challenge with the use of government procurement and AMCs to advance the deployment of clean steel is the amount of capital necessary to build a new steel plant. While a hydrogen based DRI-EAF process is likely cheaper than a full integrated BF-BOF steel mill, the overall capital costs are expected to be in excess of $1 billion (Pratty 2022). It is not clear that this mechanism can operate on the scale necessary to significantly impact deployment. For example, the Inflation Reduction Act appropriated $4.5 billion to EPA, GSA, and DOT across all construction materials (White House 2022).
Even if not sufficient by itself to drive large-scale deployment, green procurement, such as the government’s Buy Clean program, can provide a valuable source of revenue for low carbon producers. For this program, the GSA plans to develop standards for the embodied carbon in materials such as iron and steel.
Use of AMCs would require government or the private sector to commit funding to purchase low- to zero-carbon steel before the technology has been deployed. US steel consumption is on the order of 7–9 million metric tons per month. Compared with a mini-mill, which may produce only a few hundred thousand metric tons per year, an annual AMC representing less than 1 percent of US consumption would be sufficient to purchase the output of a mini-mill. Integrated steel mills, on the other hand, can produce upward of 2 million metric tons per year, which corresponds to about 2 percent of US steel consumption. This size probably keeps AMCs out of reach for these larger projects.
5.1.4.3. Standards
Since the ultimate product of all these steelmaking processes is identical to traditional steel alloys, performance standards do not seem to have a role as a demand-pull mechanism. However, standards for measuring the embodied carbon in iron and steel are useful for government procurement and AMCs. Fransen et al. (2021) pose the question of where to focus along the value chain. They recommend that the focus should be on intermediate, fabricated steel products (such as sheets, plates, bars, beams, pipes, and tubes) because of the number of promising technologies and since there is a considerably higher degree of diversity in final products making use of steel.
GSA has not yet developed standards for the embodied carbon in steel, but it issued a request for information on the subject (GSA 2022) and will likely use the results of this to develop the standards. The state of California has also set a benchmark for the greenhouse gas intensity of building materials, including steel, to be eligible for use in state-funded projects through the Buy Clean California Act.
5.1.4.4. Milestone payments
Milestone payments can be and are incorporated into DOE’s R&D funding broadly, particularly as technologies approach the pilot stage. Besides being used as a funding mechanism for basic R&D, milestone payments can be integrated into other funding mechanisms. For example, as is being done for OCED’s Hydrogen Hubs Program, demonstration funding can be given out in multiple phases, with clearly defined milestones to receive the next phase of funding. Given the use of milestone payments in the Hydrogen Hubs Program, OCED will likely use a similar model for its industrial demonstration funding going forward.
5.2. Opportunities for Application to Cement
Cement is the second-most-used material in the world after water. It is a very carbon- and energy-intensive product, responsible for 7 percent of global industrial energy usage and 2.2 billion metric tons of CO2, or 7 percent of global CO2 emissions (IEA 2018). The United States has the third-largest cement industry in the world, after China and India, producing nearly 90 million metric tons of cement in 2020 and emitting 66 million metric tons of CO2.
One of the essential steps in making ordinary Portland cement (OPC), the most common type of cement, is to heat limestone (calcium carbonate) to very high temperatures; it then forms clinker, which is mixed with crushed minerals. This process releases carbon and oxygen from the limestone, producing CO2. Finely ground clinker is a crucial binding material that reacts with water to form cement. Cement can be combined with various aggregates, including sand and stones, to form concrete. In addition to the process emissions from the limestone, the very high heat required to generate clinker is usually obtained by burning coal or other fossil fuels, which also releases CO2 (Rissman et al. 2020).
Given the unavoidable process emissions from producing cement using limestone, either those emissions must be captured and sequestered or new, alternate chemistries for making cement must be considered. In this section, we review traditional Portland cement production, substitution for clinker to make Portland cement, and alternatives to Portland cement that have lower greenhouse gas emissions.
5.2.1. Industry Overview
As of 2022, 96 cement plants were active in the United States, spread across 34 states and Puerto Rico. Texas, Missouri, California, and Florida were the leading cement-producing states, constituting 43 percent of US production in 2022 (USGS 2023). In 2017, five companies—LafargeHolcim, CEMEX, Lehigh Hanson, Buzzi Unicem USA, and Ash Grove Cement—controlled 59 percent of US cement production, which shows that the sector is fairly concentrated. On the other hand, concrete manufacturers, which use cement as their major input, are more dispersed. The cement industry has a small trade exposure of only 2 percent with the rest of the world (imports and exports over total shipments and imports) (Broekhoff et al. 2021), although interstate trade occurs, as some US states do not have cement plants within their borders.
5.2.2. Technology Background
5.2.2.1. Clinker and OPC production
Clinker is an intermediate product in the production of cement, and its manufacture generates the bulk of the CO2 emissions and energy use from cement production. Clinker is usually made in integrated cement-making facilities located near quarries.
To produce clinker, first limestone is extracted and finely crushed with other locally available minerals, such as bauxite or sand, and then these materials are preheated before entering the cement kiln. The powdered materials, or raw meal, must contain an appropriate mix of calcium carbonate, iron oxide, alumina, and silica to generate clinker. The next step is calcination, a chemical reaction that converts the limestone (calcium carbonate) into lime (calcium oxide), which will then become clinker. This chemical reaction has carbon dioxide as a byproduct. The preheated raw meal is first calcinated in a combustion chamber reaching 900°C before being completely calcinated in the rotary kiln with temperatures above 1000°C. The resulting hot clinker is then cooled by blowing air on it before storing (IEA 2018).
To create OPC, clinker is then blended and ground with other materials such as gypsum to regulate the setting time. Materials such as blast furnace slag, fly ash, or limestone can be added at this step to produce blended cement, which is more common outside the United States.
Roughly 60–70 percent of the emissions from cement production are the process emissions from the calcination process, with the remainder from the process heat needed for the precalcinator and the rotary kiln. Options for abatement in these areas include carbon capture of both emissions streams and alternative fuels for the process heat.
5.2.2.2. Substitution of other materials for clinker
Since clinker is the most carbon-intensive component of cement, the clinker-to-cement ratio is a major determinant of the cement’s carbon footprint. This ratio is particularly high in the United States compared with the rest of the world. According to Global Cement and Concrete Association and Portland Cement Association data, the average ratio is around 90 percent in the United States, while it is around 77 percent in Europe (PCA 2021).
A wide range of materials can be used as clinker substitutes in cement, but they can change the strength, setting time, and other characteristics of the resulting cement. Classic additives include blast-furnace slag and fly ash. Adding these materials has the further advantage of reusing industrial waste. These materials will likely be less abundant, however, as the industrial processes that create them are replaced by less carbon-intensive options. These additives are already commercialized, but they are not widely accepted because of constraining materials standards for buildings and roads and availability of local materials (Lehne and Preston 2018). Other raw materials, such as limestone, calcined clay, and other pozzolans, can also be used as clinker substitutes. Overall, the use of these materials to achieve a low clinker-to-cement ratio can lead to CO2 emissions reductions of more than 70 percent compared with traditional Portland cement.
5.2.2.3. Alternatives to Portland cement
Since clinker substitution does not completely eliminate the clinker or the associated process emissions from the cement, it cannot lead to a full decarbonization of cement production. To achieve this goal without carbon capture, alternative chemistries to Portland cement are needed. Four examples are alkali-activated binders, carbonatable calcium silicates, magnesium oxides, and noncarbonate calcium inputs.
Alkali-activated binders. Geopolymer cements present a promising alternative to OPC, and some are ready for commercialization. These cement products are based on alkali-activated binders replacing lime-based clinker. Geopolymer cement chemistry requires the addition of water to aluminosilicates, contained in some clays or granite, and alkaline reagents, usually sodium silicate, to form a binding mixture. Production of these binders can reduce emissions by 90 percent compared with OPC.
Carbonatable calcium silicates. New cements based on carbonatable calcium silicates sequester CO2 as they cure, which means that emissions from clinker making can be reabsorbed during the cement-curing process. Input materials are similar to the ones in OPC and are widely available. This type of cement is already commercialized by Solidia Technologies for use in pavement.
Magnesium oxides (MgO). Similar to carbonatable calcium silicates–based binders, MgO-based cements also react with CO2 during the curing process. This technology requires a large amount of natural magnesium silicate, which is widely available. This technology is still at the lab development stage but could produce carbon-negative cement.
Noncarbonate calcium inputs. The limestone used in Portland cement can be replaced with carbon-free sources of calcium such as calcium silicate, which is even more abundant than limestone. This technology has been successfully tested in lab conditions by Brimstone, which plans to build a pilot plant in 2024.
Because these alternate chemistries are unfamiliar to the construction industry, many questions about their performance will need to be answered before they can be widely deployed. To be able to replace Portland cement widely, their feedstocks must be widely available and relatively inexpensive. Some of the challenges of replacing Portland cement are discussed in Section 5.2.4.1.
5.2.3. Funding Roadmap
5.2.3.1. DOE
DOE’s Advanced Materials and Manufacturing Technologies Office (AMMTO) funds novel cement chemistries alongside other low-carbon solutions in the cement industry and other hard-to-abate sectors. In 2022, it issued an FOA targeted at projects demonstrating the production of alternative clinkers, new blended cements, and low-carbon cement production at a pilot scale that can result in more than a 50 percent reduction in carbon intensity. This FOA also seeks applications demonstrating the use of low-carbon fuels for cement and calls for projects for pilot-scale use of carbon capture (OCED 2022b).
Other DOE programs that do not specifically target the cement sector are funding a few cement-related innovation projects. ARPA-E has funded novel cement technologies under its Exploratory Topics Program, which focuses on low-TRL projects not included in its typical FOAs. Similarly, the Small Business Innovation Research Program’s topics of interest often include material efficiency innovation covering, among other things, novel cements. Nevertheless, DOE does not have a program or FOA specifically targeting novel cement chemistry. However, OCED’s Advanced Industrial Facilities Deployment Program includes cement as an area of interest.
5.2.3.2. Private sector efforts
In addition to DOE’s and other federal efforts, the Portland Cement Association (PCA), which represents a large share of the national cement industry, has released a roadmap to help the industry reach carbon neutrality by 2050, taking into account the entire value chain from quarries to built environment (PCA 2021). The roadmap highlights the need for clinker substitution and replacement solutions, increased alternative fuels and biomass in the fuel mix, and energy efficiency gains. Carbon capture technologies also play an important role in decarbonization, and PCA emphasizes the need for federal research funding for multiple carbon capture technology pilots, which will help determine the most efficient solutions for the industry and clear up uncertainties.
Regarding other policy instruments, from our interviews with industry representatives in 2021, the PCA advocates for a switch to performance-based standards in building codes and regulations, which would allow the use of already specified and commercially available low-carbon building materials (e.g., Portland limestone cement, discussed in Section 5.2.4.2). To improve market acceptance of alternatives to OPC, the government should use performance-based standards in its procurements. The industry representatives do not support the use of EPDs to determine carbon intensity thresholds for policy requirements, as these documents do not account for the cradle-to-grave life-cycle impacts, which would account for the material carbonation over time. Depending on plant size, the 45Q tax credit could also enable the development and deployment of carbon capture at cement kilns.
Global companies producing a large share of US cement, such as Heidelberg Cement, which operates Lehigh Hanson, and CEMEX, are committed to carbon neutrality by 2050 and have joined in following the PCA roadmap. Both CEMEX USA and Lehigh Hanson are developing carbon capture technologies for cement kilns in partnership with smaller innovative firms in the United States. LafargeHolcim, another major actor in the US cement industry, is developing novel chemistry cement with large emissions reductions, which will help it reach its net-zero-by-2050 commitment.
5.2.4. Applicability of Demand-Pull Mechanisms
Unlike iron and steel, where the technologies are at higher TRLs, low- to zero-carbon cement is still in the R&D stage. In this section, we explore the use of demand-pull mechanisms to drive development in this area.
5.2.4.1. Prizes
The development of a low- to zero-carbon cement has many of the characteristics that make the use of prizes beneficial. Many of the proposed alternate cements, particularly the ones that have very low to zero associated process emissions, are at early TRL stages, which means that significant R&D is needed (Lehne and Preston 2018). One requirement for a prize is a clear end product that can be stated in terms of performance characteristics rather than a particular technical solution. In this sector, the question arises whether the benchmarking of emissions should occur in cement or concrete. Fransen et al. (2021) note that it is easier to regulate cement because there are fewer production plants, the product is more uniform, and emissions reporting systems are already in place. However, this would leave out the significant potential for emissions reductions in the concrete-making stage.
Here we focus on a substitute for Portland cement. Specifying such a goal requires a detailed set of material standards that such a cement must satisfy to ensure that it can be widely substituted for current uses of cement. A specification for the amount of carbon involved in the creation of this cement is also necessary. Given the need to achieve net-zero carbon emissions by the second half of the century, we suggest that the standard for carbon emissions be a high-percentage reduction from current carbon intensities. Another choice is whether to focus on all embodied emissions in the concrete sector or on the process emissions. We suggest that the standard focus on the process emissions, since these emissions are fundamental to the creation of the cement and cannot be abated in any other manner. Emissions from process heat (discussed in Section 5.3.2) have multiple options for zero-carbon substitutes. Some proposed alternate cements absorb carbon dioxide as they cure, removing carbon from the atmosphere, and these negative emissions should be included in any carbon accounting. Finally, any such cement substitute must be deployable at scale. In particular, this means that any feedstocks into the production process must be relatively inexpensive and available in large quantities. Similarly, the price differential as compared with traditional cement, perhaps expressed in terms of an implicit carbon price, must be reasonable.
Development of prize competitions may be slow until standards are set. One possibility is that preliminary, relatively strong standards may be developed for a prize, with the hope that any product that wins the prize would be able to satisfy subsequent standards. Alternately, prizes could be awarded in stages, with a small number of winners that can satisfy the preliminary standards, followed by a second prize for satisfying the final standards, incorporating an aspect of milestone payments in the prize process.
The development of requirements for a prize is a substantial endeavor. Beyond the setting of standards, experts should come to a consensus on the requirements for cost, carbon content, and the potential for scalability. The details of the prize should also go through a public comment process, such as an RFI, as discussed in the EERE guidelines. Lastly, the value of the prize must be determined, balancing the cost to the government with the need to incentivize the research and the benefits of success. We believe that the benefits of a low-carbon, scalable cement are substantial, which would justify a relatively large prize.
5.2.4.2. Standards
Given the nearly 200-year history of Portland cement, there is substantial experience with its properties and uses. The use of any alternate cement requires confidence that it will be structurally sound and have a long life. Standards for novel cements can provide that confidence. Currently, most construction standards are prescriptive and set a specific amount of each ingredient in the cement mix. Most specify that the general-purpose OPC be made with a limited amount of clinker substitutes. These standards have been in place for decades, and they are slow to evolve and consider more advanced techniques or alternative materials (Scrivener et al. 2018).
Standards agencies must develop extensive tests and standards for the material properties of any alternate cement to ensure confidence in its use. These will likely take time to develop and will be a collaborative process with industry. The National Institute of Standards and Technology has already begun this process, with an early focus on standards for the carbon content in cement and the goal to turn later to standards for alternate cements (NIST 2022). GSA has produced standards for the carbon content in cement for government purchases (GSA 2021).
In early 2022, after working with Oregon State University to establish the safety and suitability of Portland limestone cement (PLC) for use in California, the California Department of Transportation approved the use of PLC for construction projects (Caltrans 2022). This type of cement substitutes ground limestone for some of the clinker in OPC, reducing emissions by roughly 10 percent. Standards currently exist for PLC, and it is already widely permitted in other states (PCA 2022).
5.2.4.3. Procurement and AMCs
Procurement and AMCs are both tools that could help spur the development of low- to zero-carbon cements, whereby “low- to zero-” we mean cements that almost completely eliminate their associated greenhouse gas emissions. The challenge with the use of procurement in this particular area, however, is that alternate cements with low embodied carbon are still in the R&D stage and are not expected to be viable at scale until after 2035 (DOE 2022c). Currently, there is no low- to zero-carbon cement to be procured in the United States. Once low- to zero-carbon cement is close to commercial availability, the use of government procurement will be a valuable tool to drive deployment. Nonetheless, some procurement programs exist for cement with lower carbon intensity than ordinary Portland cement.
AMCs may be applicable to demonstrate low-carbon cement technologies as they progress beyond pilot scale. While individual cement plants can be quite expensive, the overall scale of cement use in the United States means that there is likely enough capital to enable sufficiently large AMCs to be effective. A remaining challenge is whether to target particular technologies or, perhaps in the form of a prize, commit to purchasing a large quantity of any cement that achieves certain specifications including low to zero embodied carbon.
5.2.4.4. Milestone payments
R&D funding for alternate cements may be a good candidate for milestone payments. While extremely early stages of R&D may not have clear milestones, as these chemistries get closer to pilot TRLs, milestone funding could be more appropriate. In addition, as these technologies approach the demonstration scale, the discussion of milestone payments with respect to DOE demonstration funding would also apply.
5.3. Crosscutting Technologies
In this section, we look at two crosscutting technologies that are not specific to the cement and iron and steel sectors: carbon capture and fuel switching for process heat. Carbon capture is relatively mature, with multiple technologies already demonstrated or at the demonstration stage. In contrast to the general applicability of carbon capture, the characteristics of process heat vary depending on the industrial process. Many of these are at early TRLs.
Carbon capture and process heat are not products, for which there is consumer demand. Instead, they are elements in the manufacture of products that can reduce the carbon emissions produced during the manufacturing process. Thus it is not immediately clear whether a demand-pull policy for these technologies is desirable. One can certainly imagine a prize or AMC for products made using carbon capture and storage (CCS) or zero-carbon process heat, but it would likely be more efficient to instead set a standard for the carbon content of the final product and then be agnostic with respect to how the manufacturing process achieves that standard. For example, one could produce low-carbon steel either through the use of hydrogen DRI or through carbon capture on a traditional integrated steel mill. There is no a priori reason, all other things being equal, to favor one technology over the other, especially as they are at similar TRLs.
One exception to this may be milestone payments, which, because they can apply at lower TRLs, could be used for funding more nascent carbon capture and process heat technologies. That aside, 45Q is an example of a policy that directly subsidizes carbon capture rather than any final product.
Finally, it is important to recognize that opportunities exist to reduce the use of cement and iron and steel in construction, although this will not directly decarbonize either the cement or steel sector. The most prominent is the use of wood, particularly cross-laminated timber, in construction. While wood is not appropriate for many infrastructure projects, it can often be used in low- and mid-rise buildings. Many of the procurement programs for low-carbon steel and cement are also open to other low-carbon building materials such as cross-laminated timber. Note, however, that alternative materials may have a significant carbon footprint as well, so policymakers should avoid unfairly tilting the playing field against cement and steel.
5.3.1. Carbon Capture
Carbon capture is the process of removing carbon dioxide from an exhaust stream, after which it can be transported and stored underground or used in some fashion. Although there has been a significant focus on carbon capture in the electric power sector, it has been used in some form in the industrial sector for over 50 years, where the initial carbon dioxide was captured from natural gas processing and used for enhanced oil recovery. Many technologies currently exist, and NETL’s Carbon Dioxide Capture Handbook (DOE/NETL 2015) provides a useful overview. While carbon capture technologies are often classified as precombustion, postcombustion, and oxycombustion, not all emissions in the industrial sector come from combustion. Instead, these emissions come from chemical reactions that are inherent in the manufacture of products and are called process emissions. Both iron and steel and cement manufacturing include process emissions in addition to combustion emissions.
The cost to remove carbon dioxide from a process or waste stream generally decreases as the concentration of carbon dioxide in the stream increases. While the carbon dioxide concentration from an electric generator can be in the 5–10 percent range, concentrations in the industrial sector can be significantly higher because the fraction of process emissions can be so high. The cost of capture will also generally depend on the pressure of the exhaust stream. Often impurities must be removed from the stream before capture, so their type and concentration matter as well.
Common carbon capture technologies include solvents, where the carbon dioxide is dissolved in a chemical solution such as an amine; sorbents, where the carbon dioxide is absorbed or adsorbed (adhered to the surface) by a chemical; and membranes that separate the gases in the stream. Determining the most effective carbon capture solution requires matching the technologies to the particular characteristics of the exhaust streams. This may involve only the relatively pure process emissions or a combination with the combustion emissions used to generate process heat.
The Global CCS Institute (2021) has reviewed the technological readiness of various CCS technologies. Since the TRL scale it uses does not directly map to that used by DOE, we speak more generally about the readiness level rather than citing a particular TRL. By the institute’s estimation, six technologies are already at the commercial deployment stage. This includes four solvents, one sorbent, and one membrane-based technology. Thirteen more technologies have a completed, fully integrated pilot. In short, there are plenty of ready-to-deploy carbon capture technologies.
In the remainder of this section, we describe the emissions streams from the iron and steel and cement sectors and how carbon capture can work in those sectors. We then discuss the existing policy support for carbon capture and the demand-pull policies examined in this paper.
5.3.1.1. Carbon capture in the iron and steel sector
Given the complexity and multiple exhaust streams from iron and steel manufacturing, a number of different carbon capture technologies and configurations may be economic, depending on plants’ specific features (Kuramochi et al. 2012). In the iron and steel sector, for integrated mills, most of the emissions—both combustion emissions and process emissions from the reduction of iron ore with carbon—come from the blast furnaces. The resulting blast furnace gas is roughly 20 percent carbon dioxide, 20 percent carbon monoxide, and 5 percent hydrogen, with the remainder being nitrogen and water (Wiley et al. 2011). This gas could be further refined using a water-gas shift reaction to turn the carbon monoxide into carbon dioxide and hydrogen or could be recycled for use as a fuel throughout the steel mill.
The basic oxygen furnace exhaust is almost 70 percent carbon monoxide, with 15 percent carbon dioxide and 13 percent nitrogen. In terms of overall magnitude, however, the emissions from the basic oxygen furnace are over nine times less than from the blast furnace. Because of the high carbon monoxide content, the basic oxygen furnace gas can be flared or reused for energy. It could also potentially be sent through a water-gas shift reaction to generate hydrogen.
Finally, coke oven gas is roughly 50 percent hydrogen, 30 percent methane, and 7 percent carbon monoxide, with the remainder mostly carbon dioxide and nitrogen. This gas is generally burned as a source of heat for the coke oven and in other parts of the steel mill. In some mills, many of the gases are mixed and used to generate electricity. Captured exhausts can also be reformed and recirculated in the blast furnace to lower the requirements of coke as a reducing agent. ArcelorMittal is currently running a pilot plant and plans to build an industrial-scale plant in 2025 to demonstrate chemical absorption of CO2 from blast furnace flue gas.
Electric arc furnaces, which generate their heat from electricity, have low direct carbon dioxide emissions, with small amounts coming from the electrodes and some additives used in the steelmaking process. The creation of DRI will have no emissions if it is fueled solely by hydrogen, assuming the heat source is carbon free. If syngas is used, however, the carbon monoxide component will lead to carbon dioxide emissions that can be captured. Partial capture using an amine-based solvent is already in commercial use for enhanced oil recovery at the Al Reyadah plant in the United Arab Emirates. Assuming a high capture rate of 90 percent of the CO2, this technology could emit only 0.09 t CO2 per metric ton of crude steel, 94 percent less than traditional DRI-EAF (Mission Possible Partnership 2022).
5.3.1.2. Carbon capture in the cement sector
Compared with iron and steel production, the emissions from cement production are relatively simple. Roughly 60–70 percent of the total emissions are process emissions as the calcium carbonate is heated, releasing carbon dioxide, with the remainder coming from process heat (IEA 2018). Generally, both streams are combined into one exhaust stream, although there is research into having separate streams. At this point, any number of capture processes can be used. Some pretreatment of the gas to remove contaminants such as sulfur dioxide may be necessary, but this is a familiar process at electric power plants. Additional heat may also be needed to regenerate the solvent, which can be supplied in a number of different ways. Kuramochi et al. (2012) review some of the options.
Carbon capture using calcium looping has a special application to the cement sector. In this capture process, calcium oxides are used to absorb carbon dioxide, becoming calcium carbonate. The carbon dioxide is then released for transportation and utilization or storage, and the resulting calcium oxide can be reused. Calcium oxide is also the result of heating the limestone in cement manufacture. Since these chemistries overlap, either this capture process can be integrated into cement manufacturing or the calcium oxide from capture elsewhere can be used as a feedstock for cement manufacturing. The Global CCS Institute (2021) assesses these technologies to be at or near the fully integrated pilot demonstration phase. A pilot-scale demonstration was completed at a German university, and BuzziUnicem is testing this technology at a retrofitted demonstration facility through CLEANKER, a project funded by Horizon2020 running until March 2023.
5.3.1.3. Existing support for carbon capture
DOE’s Office of Fossil Energy, renamed the Office of Fossil Energy and Carbon Management in 2021, has traditionally supported carbon capture programs, with a focus on carbon capture in the electric sector. DOE’s Office of Science and Office of Energy Efficiency and Renewable Energy (EERE) also support carbon capture–related projects. Beyond DOE’s base funding for carbon capture, the IIJA includes a number of provisions that can support carbon capture (discussed in Section 4.1.1).
EERE issued an FOA that includes funding for carbon capture from cement kilns at the pilot or R&D stage (EERE 2022a). OCED and FECM issued a FOA for the Carbon Capture Demonstration Projects Program, which includes up to five carbon capture demonstration projects in industrial areas, including iron and steel and cement (OCED 2022a).
5.3.1.4. Demand-pull policies
Although policies that directly target carbon capture or zero-carbon process heat in the industrial sector are likely to be inefficient as direct mitigation policies, it may make sense to directly target early-stage technologies to reduce future costs and benefit from the spillovers from technological progress. One particular technology where that may be helpful is the use of calcium chemical looping in cements. While this technology has broad applicability, it is at an earlier TRL than many capture technologies and has a synergy with the cement sector. As such, it might be a good candidate for a targeted policy. Whereas a full cement plant can cost on the order of $1 billion, it is possible that production at a smaller scale could be targeted. Calcium looping does not seem to be a good candidate for a prize, given its midrange TRL and the likely lack of competition since the technology is already being tested at pilot scale. However, an AMC could subsidize the demonstration of this technology, and green procurement would provide a market for any cement ultimately produced. Milestone payments may make sense for funding early- to pilot-stage R&D into specific carbon capture technologies.
While demand-pull policies may not be efficient specifically for carbon capture as a component of manufacturing, these mechanisms are already being used in an adjacent technology, direct air capture (DAC). DAC is similar to carbon capture, but the concentration of CO2 in the air is much lower than in any exhaust gas stream, meaning that the technologies have some differences. However, rather than being a component in manufacturing a different product, unless the resulting carbon dioxide is used in some manner, DAC is, in a way, the final product. Thus it makes sense to target DAC with demand-pull policies, especially as there is little financial reason to capture carbon from the air without any policy driver.
Under the IIJA, DOE is in the process of establishing three prize programs with respect to DAC: the DAC Pre-Commercial Energy Program for Innovation Clusters Prize, DAC Pre-Commercial Technology Prize, and DAC Commercial Prize (DOE 2022b). These prizes collectively account for up to $115 million in funding. Each targets a different aspect of bringing this technology to market and is phased, with prizes of increasing sizes at each phase. The details of this prize process are still being determined by DOE with a tentative launch date in late February 2023.
A private AMC for carbon removal, Frontier, includes DAC. This commitment is valued at $925 million and aims to support a suite of technologies. Frontier also will fund lower-TRL technologies with prepurchase agreements. The FY2023 Omnibus Appropriations Bill also directs DOE to establish a program to purchase carbon dioxide “removed from the atmosphere or upper hydrosphere.” Depending on how this program is structured, it could be an AMC or green procurement.
All this is in addition to the direct funding of DAC by DOE, including $3.5 billion in the OCED for direct air capture hubs (OCED 2022d). This and other DOE funding falls under the auspices of DOE’s Carbon Negative Shot (FECM, n.d.).
5.3.2. Process Heat
Process heat is the delivery of heat used in industrial processes. It is extremely heterogeneous, with different processes requiring different temperatures and rates of energy delivery. Burning coal and natural gas is a carbon-intensive way of providing process heat. As an alternative to capturing the carbon dioxide from burning fossil fuels, one can seek low-carbon means of providing the process heat, such as biomass, electricity, and hydrogen. Many of the methods are reviewed in Friedmann and Tang (2019). In all situations, differences in the heat characteristics may mean that changes to plant configuration would be necessary to support switching fuels.
5.3.2.1. Iron and steel
The electric arc furnaces used in secondary and direct reduced iron steelmaking already represent a fuel substitution where electricity is used to heat the iron. In integrated steelmaking, even if the heat in the blast furnace were replaced with a low-carbon source, the process emissions stream would remain. According to Friedmann et al. (2019), given the temperatures involved, the heat could be replaced with hydrogen, biomass, or electricity. The authors do not look at the heat needs for the coke oven or the basic oxygen furnace, however. Using low-carbon heat would not eliminate the need to deal with the process carbon emissions from the coke oven, the reduction process in the blast furnace, or the basic oxygen furnace, but it could increase the concentration of carbon dioxide in the gas. In a hydrogen-based DRI, the hydrogen must be heated for the reduction reaction to occur. HYBRIT is exploring using electric resistance heating for this step (Krüger et al. 2020).
5.3.2.2. Cement
The heat needs for the production of cement are in the precalciner and the rotary kiln, with temperatures of 900°C–1250°C and 1450°C, respectively (European Commission 2020). At these temperatures, which are similar to those needed to make steel, the options are biomass, electric heating, and hydrogen. With respect to electricity, plasma generators and microwaves are still at a low TRL, and a pilot kiln running on electricity is successfully producing clinker in Finland (VTT 2023). Using hydrogen would likely require a significant modification of the kiln and is also at a low TRL. Concentrated solar power represents another low-TRL solution to the zero-carbon heat issue. These plants use large surfaces of mirrors to reflect and concentrate solar radiation in a furnace to produce industrial-grade heat.
5.3.3. Demand-Pull Policies
Demand-pull policies could target the use of low- to zero-carbon process heat in the iron and steel sector, but it is not clear why one would do that rather than more simply target the total emissions with a policy. With respect to technology-specific policies, the heterogeneity of types of process heat used across sectors complicates their use. Nonetheless, certain broadly applicable process heat technologies may be targeted. Technologies that apply only to a specific industrial process are likely more efficiently targeted by basing an award on the final carbon intensity of the process, unless there are specific technologies that are likely to have large spillovers if developed and demonstrated.
Cement production requires high temperatures for which electrification traditionally has not been suitable. However, technologies exist (Antunes et al. 2022) that could electrify the process heat in cement manufacture, leading to a relatively pure process emissions stream that is much more amenable to carbon capture. Such a technology could be targeted with milestone payments, particularly as it moves toward an integrated pilot. Similarly, a prize or AMC could be offered for cement made with electric heat. It is less clear what potential targets exist with respect to iron and steel, particularly since EAFs are already widely in use. Electric technologies such as submerged arc furnaces are being considered to enable the use of lower-purity iron ore. Procurement programs could also be targeted to particular technologies (in contrast to programs that only target the embodied carbon), but it is not clear what the advantages of such a shift would be.
6. Conclusions
With the IIJA, CHIPS, and the IRA passed by Congress giving billions of dollars to DOE in mostly grants to help industry decarbonize, the agency faces huge challenges (as do others given new responsibilities, such as for green procurement). DOE needs to staff up in a tight labor market for people knowledgeable about mitigating CO2 and how to make efficient decisions. Yet potential recipients of DOE funding, even with all the cash flowing and subsidies, may with good reason be reluctant to propose and promise success for massive projects with unproven (at scale) technologies. With cost shares accompanying these grants, the private actors with enough cash to self-finance may be few and may favor a set of solutions that enable their core business to proceed as before. External finance may be hard to come by as well, given the financial, technological, and managerial risks.
It is against this backdrop that we ask whether nontraditional, demand-pull innovation policies can help. Certainly, by focusing on growing demand, these policies can reduce offtake risks, making private capital financing a better bet. And pairing traditional supply-side tools with complementary demand-side tools can further reduce the risks as evaluated by potential applicants and external funders.
However, these tools will not implement themselves. Green procurement, for instance, requires carbon intensity benchmarks, environmental product declarations, and decisionmaking tools, which need knowledgeable staff and the time and resources to learn how to design and use them. Early failures, in the face of all the pressure to succeed quickly, should be expected and tolerated. Legal and administrative barriers may limit application of some of the tools we reviewed, although the relevant agencies have used some types of these tools before. Ultimately, greater reliance on these demand-pull tools necessitates high-level administrative commitment and perhaps a willingness to be patient.
As with any subsidy, there is a risk of subsidizing things that would occur otherwise. For example, many projects are in the works for adding carbon capture technology to existing ethanol plants, even under a much less generous subsidy than the one offered in the IRA (Douglas 2022). If these projects apply for and receive the more generous subsidy ($85 per metric ton of CO2 stored versus $50 per metric ton), the difference is a waste of taxpayer money and an inefficient use of the resources this money could otherwise employ. To the extent that a government subsidy exists to promote a positive externality or mitigate a negative one, however, this is less of a concern from a societal point of view. In fact, the subsidy acts to appropriately reward the recipient in such a way that better reflects the societal impact of its investment. In the case of the subsidies discussed here, whether supply push or demand pull, they seek to remedy both the spillover benefits of knowledge diffusion from R&D and the fact that the damages from climate change are not priced.
Overall, the carrots of subsidies, as opposed to the sticks of regulation, present a difficult challenge to the government in balancing the altogether justifiable act of mitigating negative externalities with the possibility that some of the funds will be wasted. In this case, the urgency for technological progress on and market penetration of climate mitigating technologies, in the midst of congressional roadblocks to all but subsidies, argues for erring on the side of addressing climate change.
Most demand-pull tools are currently being applied by the federal government. DOE has increased its use of prizes, although far more could be done where low-TRL technologies are still being developed. Milestone payments also are seeing a boost in use, such as for the Hydrogen Hubs Program, which has multiple phases, and they can be combined with various supply-push policies to add some aspects of demand pull, such as for demonstration projects. The private sector is beginning to use AMCs for carbon mitigation (particularly direct air capture), suggesting that the pressures being exerted by shareholders, rating agencies, and others on companies to make CO2 reduction pledges, and to make good on them, can simultaneously take some pressure off governments. The significant augmentation of green procurement policies toward road, bridge, and other construction projects using green steel and cement, among other materials, is most promising, as government demand is so large, particularly considering the Federal Highway Trust Fund. The 2023 budget also includes a direction for DOE to begin to procure carbon from direct air capture. Moreover, the 2023 RFI from OCED at DOE strongly indicates that the office plans to implement more demand-side measures as part of its funding for clean energy technologies (OCED 2023).
In terms of specific tools applicable to specific sectors (iron and steel and cement), we find limited applicability for the other demand-pull instruments. Technologies to decarbonize iron and steel are already being demonstrated, which makes prizes less applicable, and even relatively nascent technologies, such as electrolysis, are at the pilot stage. In addition, the large amount of money involved in a new iron and steel plant makes AMCs challenging. Still, green procurement of projects, such as roads and bridges, that mandate the use of green steel will reduce costs and provide an incentive for demonstration projects.
The situation for cement is different because few, if any, zero-carbon cement options are at high TRLs (with the exception of the use of carbon capture). A number of high-TRL cements reduce the carbon emissions associated with cement production, but none are sufficient to achieve net-zero emissions in the sector. However, some new chemistries, still at the low-TRL stage, could be developed into replacements for Portland cement and eliminate any associated emissions. We believe that this is a prime opportunity for the use of a prize to motivate further R&D of these materials. Standards will also be an important part of the success of any new chemistries to ensure that they can be used by the private sector, reducing any concerns that might be associated with a new material being used in essential infrastructure. Finally, as new cements move closer to market, green procurement and even AMCs can be used to help drive employment, especially combined with standards for their embodied carbon.
There are also broadly applicable technologies that can serve to reduce emissions from the iron and steel and cement sectors. We examined the case of carbon capture and process heat. Because these are component technologies, demand-pull methods may not be as efficient as those targeting a final product. Nonetheless, it may make sense to target specific technologies that have unique synergies with a demand-pull policy, such as calcium looping carbon capture in the cement sector. Applying demand-pull methods to these crosscutting technologies also must be done in the context of tax credit programs already in place for CCUS and hydrogen (both blue and green).
Finally, demand-pull and supply-push innovation policies inherently pick winners at some level and will be less efficient than policies that cut across sectors and provide medium- and long-term incentives to reduce greenhouse gas emissions. Such policies as carbon taxes, tradable performance standards, and other regulatory approaches have been off the table legislatively. But they need their day in the sun as well.
Authors
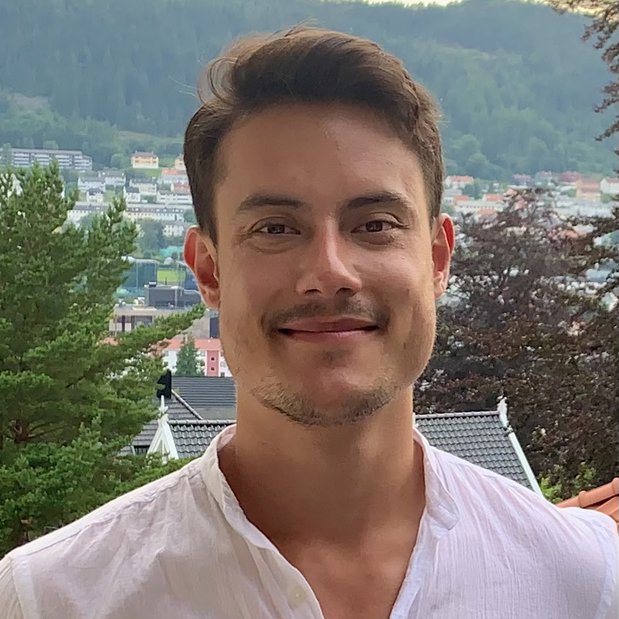
Daniel Haerle
VU Amsterdam